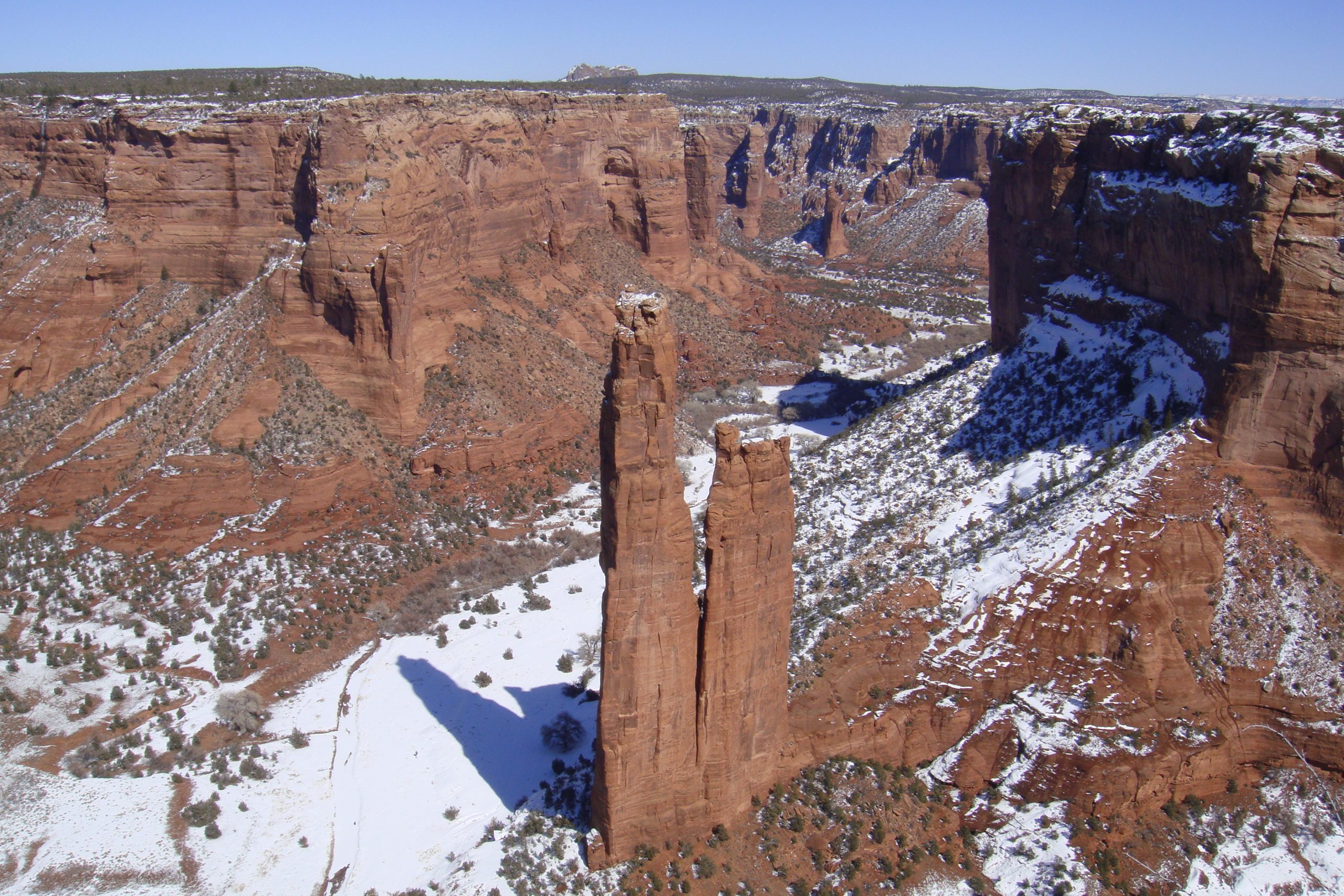
KEY CONCEPTS
By the end of this chapter, students should be able to:
- Explain the big-bang theory and origin of the elements
- Explain the solar system’s origin and the consequences for Earth.
- Describe the turbulent beginning of Earth during the Hadean and Archean Eons
- Identify the transition to modern atmosphere, plate tectonics, and evolution that occurred in the Proterozoic Eon
- Describe the Paleozoic evolution and extinction of invertebrates with hard parts, fish, amphibians, reptiles, tetrapods, and land plants; and tectonics and sedimentation associated with the supercontinent Pangea
- Describe the Mesozoic evolution and extinction of birds, dinosaurs, and mammmals; and tectonics and sedimentation associated with the breakup of Pangea
- Describe the Cenozoic evolution of mammals and birds, paleoclimate, and tectonics that shaped the modern world
Entire courses and careers have been based on the wide-ranging topics covering Earth’s history. Throughout the long history of Earth, change has been the norm. Looking back in time, an untrained eye would see many unfamiliar life forms and terrains. The main topics studied in Earth history are paleogeography, paleontology, and paleoecology and paleoclimatology—respectively, past landscapes, past organisms, past ecosystems, and past environments. This chapter will cover briefly the origin of the universe and the 4.6 billion year history of Earth. This Earth history will focus on the major physical and biological events in each Eons and Era.
8.1 Origin of the Universe
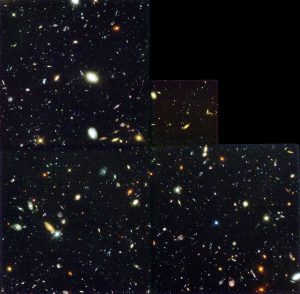
The universe appears to have an infinite number of galaxies and solar systems and our solar system occupies a small section of this vast entirety. The origins of the universe and solar system set the context for conceptualizing the Earth’s origin and early history.
8.1.1 Big-Bang Theory
The mysterious details of events prior to and during the origin of the universe are subject to great scientific debate. The prevailing idea about how the universe was created is called the big-bang theory. Although the ideas behind the big-bang theory feel almost mystical, they are supported by Einstein’s theory of general relativity. Other scientific evidence, grounded in empirical observations, supports the big-bang theory.
The big-bang theory proposes the universe was formed from an infinitely dense and hot core of material. The bang in the title suggests there was an explosive, outward expansion of all matter and space that created atoms. Spectroscopy confirms that hydrogen makes up about 74% of all matter in the universe. Since its creation, the universe has been expanding for 13.8 billion years and recent observations suggest the rate of this expansion is increasing.
Spectroscopy
Spectroscopy is the investigation and measurement of spectra produced when materials interacts with or emits electromagnetic radiation. Spectra is the plural for spectrum which is a particular wavelength from the electromagnetic spectrum. Common spectra include the different colors of visible light, X-rays, ultraviolet waves, microwaves, and radio waves. Each beam of light is a unique mixture of wavelengths that combine across the spectrum to make the color we see. The light wavelengths are created or absorbed inside atoms, and each wavelength signature matches a specific element. Even white light from the Sun, which seems like an uninterrupted continuum of wavelengths, has gaps in some wavelengths. The gaps correspond to elements present in the Earth’s atmosphere that act as filters for specific wavelengths. These missing wavelengths were famously observed by Joseph von Fraunhofer (1787–1826) in the early 1800s, but it took decades before scientists were able to relate the missing wavelengths to atmospheric filtering. Spectroscopy shows that the Sun is mostly made of hydrogen and helium. Applying this process to light from distant stars, scientists can calculate the abundance of elements in a specific star and visible universe as a whole. Also, this spectroscopic information can be used as an interstellar speedometer.
Redshift
The Doppler effect is the same process that changes the pitch of the sound of an approaching car or ambulance from high to low as it passes. When an object emits waves, such as light or sound, while moving toward an observer, the wavelengths get compressed. In sound, this results in a shift to a higher pitch. When an object moves away from an observer, the wavelengths are extended, producing a lower pitched sound. The Doppler effect is used on light emitted from stars and galaxies to determine their speed and direction of travel. Scientists, including Vesto Slipher (1875–1969) and Edwin Hubble (1889–1953), examined galaxies both near and far and found that almost all galaxies outside of our galaxy are moving away from each other, and us. Because the light wavelengths of receding objects are extended, visible light is shifted toward the red end of the spectrum, called a redshift. In addition, Hubble noticed that galaxies that were farther away from Earth also had the greater amount of redshift, and thus, the faster they are traveling away from us. The only way to reconcile this information is to deduce the universe is still expanding. Hubble’s observation forms the basis of big-bang theory.
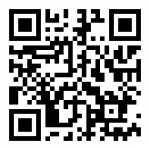
Cosmic Microwave Background Radiation
Another strong indication of the big-bang is cosmic microwave background radiation. Cosmic radiation was accidentally discovered by Arno Penzias (1933–) and Robert Woodrow Wilson (1936–) when they were trying to eliminate background noise from a communication satellite. They discovered very faint traces of energy or heat that are omnipresent across the universe. This energy was left behind from the big bang, like an echo.
8.1.2 Stellar Evolution
Astronomers think the big bang created lighter elements, mostly hydrogen and smaller amounts of elements helium, lithium, and beryllium. Another process must be responsible for creating the other 90 heavier elements. The current model of stellar evolution explains the origins of these heavier elements.
Birth of a star
Stars start their lives as elements floating in cold, spinning clouds of gas and dust known as nebulas. Gravitational attraction or perhaps a nearby stellar explosion causes the elements to condense and spin into disk shape. In the center of this disk shape a new star is born under the force of gravity. The spinning whirlpool concentrates material in the center, and the increasing gravitational forces collect even more mass. Eventually, the immensely concentrated mass of material reaches a critical point of such intense heat and pressure it initiates fusion.
Fusion
Fusion is not a chemical reaction. Fusion is a nuclear reaction in which two or more nuclei, the centers of atoms, are forced together and combine creating a new larger atom. This reaction gives off a tremendous amount of energy, usually as light and solar radiation. An element such as hydrogen combines or fuses with other hydrogen atoms in the core of a star to become a new element, in this case, helium. Another product of this process is energy, such as solar radiation that leaves the Sun and comes to the Earth as light and heat. Fusion is a steady and predictable process, which is why we call this the main phase of a star’s life. During its main phase, a star turns hydrogen into helium. Since most stars contain plentiful amounts of hydrogen, the main phase may last billions of years, during which their size and energy output remains relatively steady.
The giant phase in a star’s life occurs when the star runs out of hydrogen for fusion. If a star is large enough, it has sufficient heat and pressure to start fusing helium into heavier elements. This style of fusion is more energetic and the higher energy and temperature expand the star to a larger size and brightness. This giant phase is predicted to happen to our Sun in another few billion years, growing the radius of the Sun to Earth’s orbit, which will render life impossible. The mass of a star during its main phase is the primary factor in determining how it will evolve. If the star has enough mass and reaches a point at which the primary fusion element, such as helium, is exhausted, fusion continues using new, heavier elements. This occurs over and over in very large stars, forming progressively heavier elements like carbon and oxygen. Eventually, fusion reaches its limit as it forms iron and nickel. This progression explains the abundance of iron and nickel in rocky objects, like Earth, within the solar system. At this point, any further fusion absorbs energy instead of giving it off, which is the beginning of the end of the star’s life.
Death of a Star
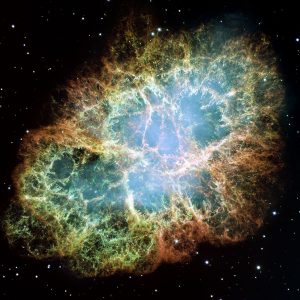
The death of a star can range from spectacular to other-worldly (see figure). Stars like the Sun form a planetary nebula, which comes from the collapse of the star’s outer layers in an event like the implosion of a building. In the tug-of-war between gravity’s inward pull and fusion’s outward push, gravity instantly takes over when fusion ends, with the outer gasses puffing away to form a nebula. More massive stars do this as well but with a more energetic collapse, which starts another type of energy release mixed with element creation known as a supernova. In a supernova, the collapse of the core suddenly halts, creating a massive outward-propagating shock wave. A supernova is the most energetic explosion in the universe short of the big bang. The energy release is so significant the ensuing fusion can make every element up through uranium.
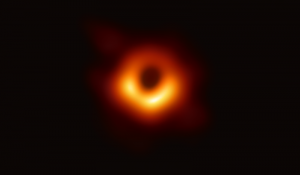
The death of the star can result in the creation of white dwarfs, neutron stars, or black holes. Following their deaths, stars like the Sun turn into white dwarfs.
White dwarfs are hot star embers, formed by packing most of a dying star’s mass into a small and dense object about the size of Earth. Larger stars may explode in a supernova that packs their mass even tighter to become neutron stars. Neutron stars are so dense that protons combine with electrons to form neutrons. The largest stars collapse their mass even further, becoming objects so dense that light cannot escape their gravitational grasp. These are the infamous black holes and the details of the physics of what occurs in them are still up for debate.
Take this quiz to check your comprehension of this section.
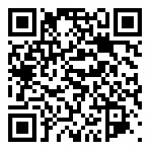
8.2 Origin of the Solar System: The Nebular Hypothesis
Our solar system formed at the same time as our Sun as described in the nebular hypothesis. The nebular hypothesis is the idea that a spinning cloud of dust made of mostly light elements, called a nebula, flattened into a protoplanetary disk, and became a solar system consisting of a star with orbiting planets. The spinning nebula collected the vast majority of material in its center, which is why the sun Accounts for over 99% of the mass in our solar system.
8.2.1 Planet Arrangement and Segregation
As our solar system formed, the nebular cloud of dispersed particles developed distinct temperature zones. Temperatures were very high close to the center, only allowing condensation of metals and silicate minerals with high melting points. Farther from the Sun, the temperatures were lower, allowing the condensation of lighter gaseous molecules such as methane, ammonia, carbon dioxide, and water. This temperature differentiation resulted in the inner four planets of the solar system becoming rocky, and the outer four planets becoming gas giants.
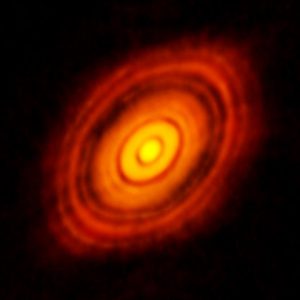
Both rocky and gaseous planets have a similar growth model. Particles of dust, floating in the disc were attracted to each other by static charges and eventually, gravity. As the clumps of dust became bigger, they interacted with each other—colliding, sticking, and forming proto-planets. The planets continued to grow over the course of many thousands or millions of years, as material from the protoplanetary disc was added. Both rocky and gaseous planets started with a solid core. Rocky planets built more rock on that core, while gas planets added gas and ice. Ice giants formed later and on the furthest edges of the disc, accumulating less gas and more ice. That is why the gas-giant planets Jupiter and Saturn are composed of mostly hydrogen and helium gas, more than 90%. The ice giants Uranus and Neptune are composed of mostly methane ices and only about 20% hydrogen and helium gases.
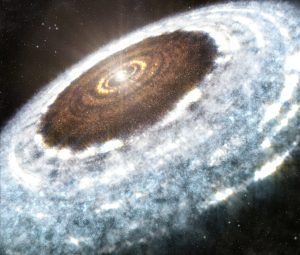
The planetary composition of the gas giants is clearly different from the rocky planets. Their size is also dramatically different for two reasons: First, the original planetary nebula contained more gases and ices than metals and rocks. There was abundant hydrogen, carbon, oxygen, nitrogen, and less silicon and iron, giving the outer planets more building material. Second, the stronger gravitational pull of these giant planets allowed them to collect large quantities of hydrogen and helium, which could not be collected by weaker gravity of the smaller planets.
Jupiter’s massive gravity further shaped the solar system and growth of the inner rocky planets. As the nebula started to coalesce into planets, Jupiter’s gravity accelerated the movement of nearby materials, generating destructive collisions rather than constructively gluing material together. These collisions created the asteroid belt, an unfinished planet, located between Mars and Jupiter. This asteroid belt is the source of most meteorites that currently impact the Earth. Study of asteroids and meteorites help geologist to determine the age of Earth and the composition of its core, mantle, and crust. Jupiter’s gravity may also explain Mars’ smaller mass, with the larger planet consuming material as it migrated from the inner to outer edge of the solar system.
Pluto and planet definition
The outermost part of the solar system is known as the Kuiper belt, which is a scattering of rocky and icy bodies. Beyond that is the Oort cloud, a zone filled with small and dispersed ice traces. These two locations are where most comets form and continue to orbit, and objects found here have relatively irregular orbits compared to the rest of the solar system. Pluto, formerly the ninth planet, is located in this region of space. The XXVIth General Assembly of the International Astronomical Union (IAU) stripped Pluto of planetary status in 2006 because scientists discovered an object more massive than Pluto, which they named Eris. The IAU decided against including Eris as a planet, and therefore, excluded Pluto as well. The IAU narrowed the definition of a planet to three criteria: 1) enough mass to have gravitational forces that force it to be rounded, 2) not massive enough to create fusion, and 3) large enough to be in a cleared orbit, free of other planetesimals that should have been incorporated at the time the planet formed. Pluto passed the first two parts of the definition, but not the third. Pluto and Eris are currently classified as dwarf planets.
Take this quiz to check your comprehension of this section.
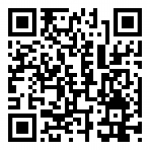
8.3 Hadean Eon
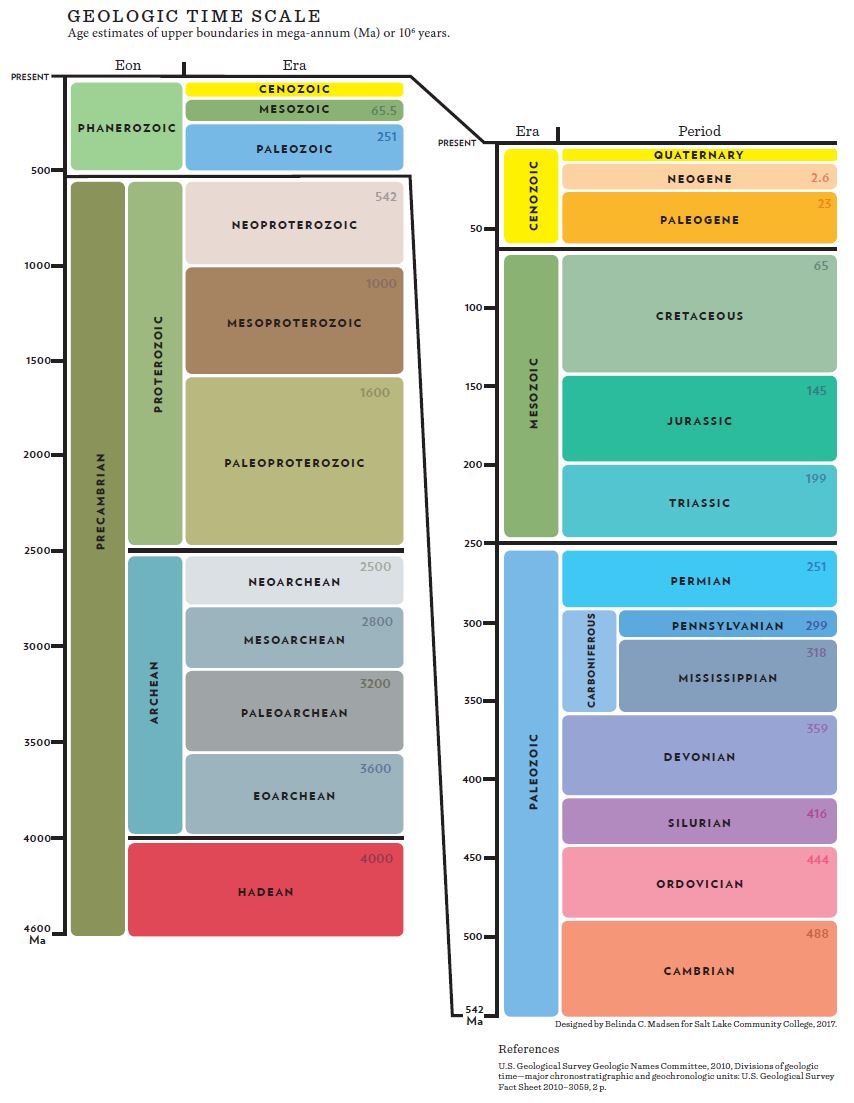
Geoscientists use the geological time scale to assign relative age names to events and rocks, separating major events in Earth’s history based on significant changes as recorded in rocks and fossils. This section summarizes the most notable events of each major time interval. For a breakdown on how these time intervals are chosen and organized, see chapter 7.
The Hadean Eon, named after the Greek god and ruler of the underworld Hades, is the oldest eon and dates from 4.5–4.0 billion years ago.
This time represents Earth’s earliest history, during which the planet was characterized by a partially molten surface, volcanism, and asteroid impacts. Several mechanisms made the newly forming Earth incredibly hot: gravitational compression, radioactive decay, and asteroid impacts. Most of this initial heat still exists inside the Earth. The Hadean was originally defined as the birth of the planet occurring 4.0 billion years ago and preceding the existence of many rocks and life forms. However, geologists have dated minerals at 4.4 billion years, with evidence that liquid water was present. There is possibly even evidence of life existing over 4.0 billion years ago. However, the most reliable record for early life, the microfossil record, starts at 3.5 billion years ago.
8.3.1 Origin of Earth’s Crust
As Earth cooled from its molten state, minerals started to crystallize and settle resulting in a separation of minerals based on density and the creation of the crust, mantle, and core. The earliest Earth was chiefly molten material and would have been rounded by gravitational forces so it resembled a ball of lava floating in space. As the outer part of the Earth slowly cooled, the high melting-point minerals (see Bowen’s Reaction Series in Chapter 4) formed solid slabs of early crust. These slabs were probably unstable and easily reabsorbed into the liquid magma until the Earth cooled enough to allow numerous larger fragments to form a thin primitive crust. Scientists generally assume this crust was oceanic and mafic in composition, and littered with impacts, much like the Moon’s current crust. There is still some debate over when plate tectonics started, which would have led to the formation of continental and felsic crust. Regardless of this, as Earth cooled and solidified, less dense felsic minerals floated to the surface of the Earth to form the crust, while the denser mafic and ultramafic materials sank to form the mantle and the highest-density iron and nickel sank into the core. This differentiated the Earth from a homogenous planet into a heterogeneous one with layers of felsic crust, mafic crust, ultramafic mantle, and iron and nickel core.
8.3.2 Origin of the Moon
Several unique features of Earth’s Moon have prompted scientists to develop the current hypothesis about its formation. The Earth and Moon are tidally locked, meaning that as the Moon orbits, one side always faces the Earth and the opposite side is not visible to us. Also and most importantly, the chemical compositions of the Earth and Moon show nearly identical isotope ratios and volatile content. Apollo missions returned from the Moon with rocks that allowed scientists to conduct very precise comparisons between Moon and Earth rocks. Other bodies in the solar system and meteorites do not share the same degree of similarity and show much higher variability. If the Moon and Earth formed together, this would explain why they are so chemically similar.
Many ideas have been proposed for the origin of the Moon: The Moon could have been captured from another part of the solar system and formed in place together with the Earth, or the Moon could have been ripped out of the early Earth. None of proposed explanations can account for all the evidence. The currently prevailing hypothesis is the giant-impact hypothesis. It proposes a body about half of Earth’s size must have shared at least parts of Earth’s orbit and collided with it, resulting in a violent mixing and scattering of material from both objects. Both bodies would be composed of a combination of materials, with more of the lower density splatter coalescing into the Moon. This may explain why the Earth has a higher density and thicker core than the Moon.
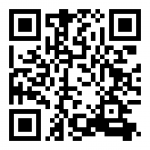
Computer simulation of the evolution of the Moon (2 minutes).
8.3.3 Origin of Earth’s Water
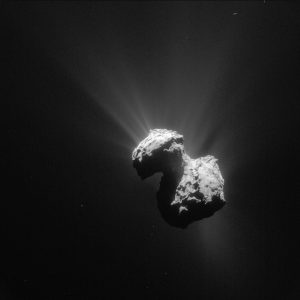
Explanations for the origin of Earth’s water include volcanic outgassing, comets, and meteorites. The volcanic outgassing hypothesis for the origin of Earth’s water is that it originated from inside the planet, and emerged via tectonic processes as vapor associated with volcanic eruptions. Since all volcanic eruptions contain some water vapor, at times more than 1% of the volume, these alone could have created Earth’s surface water. Another likely source of water was from space. Comets are a mixture of dust and ice, with some or most of that ice being frozen water. Seemingly dry meteors can contain small but measurable amounts of water, usually trapped in their mineral structures. During heavy bombardment periods later in Earth’s history, its cooled surface was pummeled by comets and meteorites, which could be why so much water exists above ground. There isn’t a definitive answer for what process is the source of ocean water. Earth’s water isotopically matches water found in meteorites much better than that of comets. However, it is hard to know if Earth processes could have changed the water’s isotopic signature over the last 4-plus billion years. It is possible that all three sources contributed to the origin of Earth’s water.
Take this quiz to check your comprehension of this section.
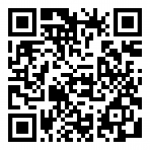
8.4 Archean Eon
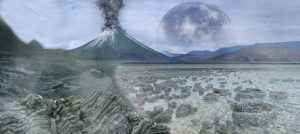
The Archean Eon, which lasted from 4.0–2.5 billion years ago, is named after the Greek word for beginning. This eon represents the beginning of the rock record. Although there is current evidence that rocks and minerals existed during the Hadean Eon, the Archean has a much more robust rock and fossil record.
8.4.1 Late Heavy Bombardment
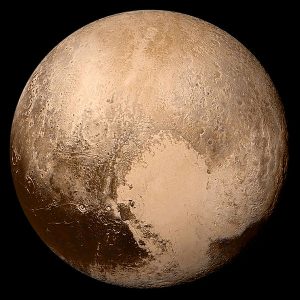
Objects were chaotically flying around at the start of the solar system, building the planets and moons. There is evidence that after the planets formed, about 4.1–3.8 billion years ago, a second large spike of asteroid and comet impacted the Earth and Moon in an event called late heavy bombardment. Meteorites and comets in stable or semi-stable orbits became unstable and started impacting objects throughout the solar system. In addition, this event is called the lunar cataclysm because most of the Moons craters are from this event. During late heavy bombardment, the Earth, Moon, and all planets in the solar system were pummeled by material from the asteroid and Kuiper belts. Evidence of this bombardment was found within samples collected from the Moon.
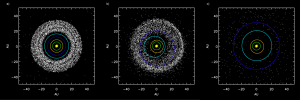
It is universally accepted that the solar system experienced extensive asteroid and comet bombardment at its start; however, some other process must have caused the second increase in impacts hundreds of millions of years later. A leading theory blames gravitational resonance between Jupiter and Saturn for disturbing orbits within the asteroid and Kuiper belts based on a similar process observed in the Eta Corvi star system.
8.4.2 Origin of the Continents
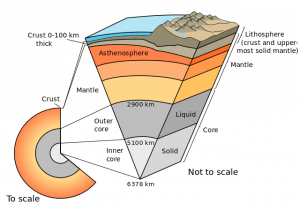
In order for plate tectonics to work as it does currently, it necessarily must have continents. However, the easiest way to create continental material is via assimilation and differentiation of existing continents (see Chapter 4). This chicken-and-egg quandary over how continents were made in the first place is not easily answered because of the great age of continental material and how much evidence has been lost during tectonics and erosion. While the timing and specific processes are still debated, volcanic action must have brought the first continental material to the Earth’s surface during the Hadean, 4.4 billion years ago. This model does not solve the problem of continent formation, since magmatic differentiation seems to need thicker crust. Nevertheless, the continents formed by some incremental process during the early history of Earth. The best idea is that density differences allowed lighter felsic materials to float upward and heavier ultramafic materials and metallic iron to sink. These density differences led to the layering of the Earth, the layers that are now detected by seismic studies. Early protocontinents accumulated felsic materials as developing plate–tectonic processes brought lighter material from the mantle to the surface.
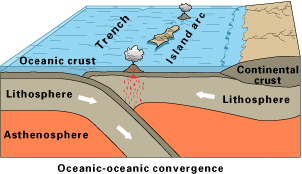
The first solid evidence of modern plate tectonics is found at the end of the Archean, indicating at least some continental lithosphere must have been in place. This evidence does not necessarily mark the starting point of plate tectonics; remnants of earlier tectonic activity could have been erased by the rock cycle.
The stable interiors of the current continents are called cratons and were mostly formed in the Archean Eon. A craton has two main parts: the shield, which is crystalline basement rock near the surface, and the platform made of sedimentary rocks covering the shield. Most cratons have remained relatively unchanged with most tectonic activity having occurred around cratons instead of within them. Whether they were created by plate tectonics or another process, Archean continents gave rise to the Proterozoic continents that now dominate our planet.
The general guideline as to what constitutes a continent and differentiates oceanic from continental crust is under some debate. At passive margins, continental crust grades into oceanic crust at passive margins, making a distinction difficult. Even island-arc and hot-spot material can seem more closely related to continental crust than oceanic. Continents usually have a craton in the middle with felsic igneous rocks. There is evidence that submerged masses like Zealandia, that includes present-day New Zealand, would be considered a continent. Continental crust that does not contain a craton is called a continental fragment, such as the island of Madagascar off the east coast of Africa.
8.4.3 First Life on Earth
Life most likely started during the late Hadean or early Archean Eons. The earliest evidence of life are chemical signatures, microscopic filaments, and microbial mats. Carbon found in 4.1 billion year old zircon grains have a chemical signature suggesting an organic origin. Other evidence of early life are 3.8–4.3 billion-year-old microscopic filaments from a hydrothermal vent deposit in Quebec, Canada. While the chemical and microscopic filaments evidence is not as robust as fossils, there is significant fossil evidence for life at 3.5 billion years ago. These first well-preserved fossils are photosynthetic microbial mats, called stromatolites, found in Australia.
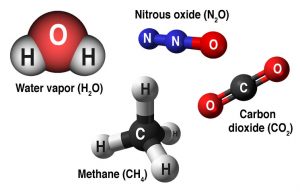
Although the origin of life on Earth is unknown, hypotheses include a chemical origin in the early atmosphere and ocean, deep-sea hydrothermal vents, and delivery to Earth by comets or other objects. One hypothesis is that life arose from the chemical environment of the Earth’s early atmosphere and oceans, which was very different than today. The oxygen-free atmosphere produced a reducing environment with abundant methane, carbon dioxide, sulfur, and nitrogen compounds. This is what the atmosphere is like on other bodies in the solar system. In the famous Miller-Urey experiment, researchers simulated early Earth’s atmosphere and lightning within a sealed vessel. After igniting sparks within the vessel, they discovered the formation of amino acids, the fundamental building blocks of proteins. In 1977, when scientists discovered an isolated ecosystem around hydrothermal vents on a deep-sea mid-ocean ridge (see Chapter 4), it opened the door for another explanation of the origin of life. The hydrothermal vents have a unique ecosystem of critters with chemosynthesis as the foundation of the food chain instead of photosynthesis. The ecosystem is deriving its energy from hot chemical-rich waters pouring out of underground towers. This suggests that life could have started on the deep ocean floor and derived energy from the heat from the Earth’s interior via chemosynthesis. Scientists have since expanded the search for life to more unconventional places, like Jupiter’s icy moon Europa.
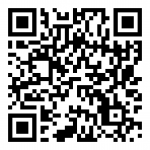
Animation of the original Miller-Urey 1959 experiment that simulated the early atmosphere and created amino acids from simple elements and compounds.
Another possibility is that life or its building blocks came to Earth from space, carried aboard comets or other objects. Amino acids, for example, have been found within comets and meteorites. This intriguing possibility also implies a high likelihood of life existing elsewhere in the cosmos.
Take this quiz to check your comprehension of this section.
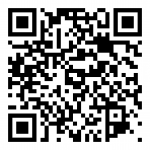
8.5 Proterozoic Eon
The Proterozoic Eon, meaning “earlier life,” comes after the Archean Eon and ranges from 2.5 billion to 541 million years old. During this time, most of the central parts of the continents had formed and plate tectonic processes had started. Photosynthesis by microbial organisms, such as single-celled cyanobacteria, had been slowly adding oxygen to the oceans. As cyanobacteria evolved into multicellular organisms, they completely transformed the oceans and later the atmosphere by adding massive amounts of free oxygen gas (O2) and initiated what is called the Great Oxygenation Event (GOE). This drastic environmental change decimated the anaerobic bacteria, which could not survive in the presence of free oxygen. On the other hand, aerobic organisms could thrive in ways they could not earlier.
An oxygenated world also changed the chemistry of the planet in significant ways. For example, iron remained in solution in the non-oxygenated environment of the earlier Archean Eon. In chemistry, this is known as a reducing environment. Once the environment was oxygenated, iron combined with free oxygen to form solid precipitates of iron oxide, such as the mineral hematite or magnetite. These precipitates accumulated into large mineral deposits with red chert known as banded-iron formations, which are dated at about 2 billion years.
The formation of iron oxide minerals and red chert (see figure) in the oceans lasted a long time and prevented oxygen levels from increasing significantly, since precipitation took the oxygen out of the water and deposited it into the rock strata. As oxygen continued to be produced and mineral precipitation leveled off, dissolved oxygen gas eventually saturated the oceans and started bubbling out into the atmosphere. Oxygenation of the atmosphere is the single biggest event that distinguishes the Archean and Proterozoic environments. In addition to changing mineral and ocean chemistry, the GOE is also tabbed as triggering Earth’s first glaciation event around 2.1 billion years ago, the Huron Glaciation. Free oxygen reacted with methane in the atmosphere to produce carbon dioxide. Carbon dioxide and methane are called greenhouse gases because they trap heat within the Earth’s atmosphere, like the insulated glass of a greenhouse. Methane is a more effective insulator than carbon dioxide, so as the proportion of carbon dioxide in the atmosphere increased, the greenhouse effect decreased, and the planet cooled.
8.5.1 Rodinia
By the Proterozoic Eon, lithospheric plates had formed and were moving according to plate tectonic forces that were similar to current times. As the moving plates collided, the ocean basins closed to form a supercontinent called Rodinia. The supercontinent formed about 1 billion years ago and broke up about 750 to 600 million years ago, at the end of the Proterozoic. One of the resulting fragments was a continental mass called Laurentia that would later become North America. Geologists have reconstructed Rodinia by matching and aligning ancient mountain chains, assembling the pieces like a jigsaw puzzle, and using paleomagnetics to orient to magnetic north.
The disagreements over these complex reconstructions is exemplified by geologists proposing at least six different models for the breakup of Rodinia to create Australia, Antarctica, parts of China, the Tarim craton north of the Himalaya, Siberia, or the Kalahari craton of eastern Africa. This breakup created lots of shallow-water, biologically favorable environments that fostered the evolutionary breakthroughs marking the start of the next eon, the Phanerozoic.
8.5.2 Life Evolves
Early life in the Archean and earlier is poorly documented in the fossil record. Based on chemical evidence and evolutionary theory, scientists propose this life would have been single-celled photosynthetic organisms, such as the cyanobacteria that created stromatolites. Cyanobacteria produced free oxygen in the atmosphere through photosynthesis. Cyanobacteria, archaea, and bacteria are prokaryotes—primitive organisms made of single cells that lack cell nuclei and other organelles.
A large evolutionary step occurred during the Proterozoic Eon with the appearance of eukaryotes around 2.1 to 1.6 billion years ago. Eukaryotic cells are more complex, having nuclei and organelles. The nuclear DNA is capable of more complex replication and regulation than that of prokaryotic cells. The organelles include mitochondria for producing energy and chloroplasts for photosynthesis. The eukaryote branch in the tree of life gave rise to fungi, plants, and animals.
Another important event in Earth’s biological history occurred about 1.2 billion years ago when eukaryotes invented sexual reproduction. Sharing genetic material from two reproducing individuals, male and female, greatly increased genetic variability in their offspring. This genetic mixing accelerated evolutionary change, contributing to more complexity among individual organisms and within ecosystems (see Chapter 7).
Proterozoic land surfaces were barren of plants and animals and geologic processes actively shaped the environment differently because land surfaces were not protected by leafy and woody vegetation. For example, rain and rivers would have caused erosion at much higher rates on land surfaces devoid of plants. This resulted in thick accumulations of pure quartz sandstone from the Proterozoic Eon such as the extensive quartzite formations in the core of the Uinta Mountains in Utah.
Fauna during the Ediacaran Period, 635.5 to 541 million years ago are known as the Ediacaran fauna, and offer a first glimpse at the diversity of ecosystems that evolved near the end of the Proterozoic. These soft-bodied organisms were among the first multicellular life forms and probably were similar to jellyfish or worm-like. Ediacaran fauna did not have hard parts like shells and were not well preserved in the rock records. However, studies suggest they were widespread in the Earth’s oceans. Scientists still debate how many species were evolutionary dead-ends that became extinct and how many were ancestors of modern groupings. The transition of soft-bodied Ediacaran life to life forms with hard body parts occurred at the end of the Proterozoic and beginning of the Phanerozoic Eons. This evolutionary explosion of biological diversity made a dramatic difference in scientists’ ability to understand the history of life on Earth.
Take this quiz to check your comprehension of this section.
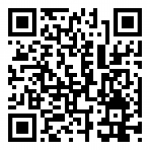
8.6 Phanerozoic Eon: Paleozoic Era
The Phanerozoic Eon is the most recent, 541 million years ago to today, and means “visible life” because the Phanerozoic rock record is marked by an abundance of fossils. Phanerozoic organisms had hard body parts like claws, scales, shells, and bones that were more easily preserved as fossils. Rocks from the older Precambrian time are less commonly found and rarely include fossils because these organisms had soft body parts. Phanerozoic rocks are younger, more common, and contain the majority of extant fossils. The study of rocks from this eon yields much greater detail. The Phanerozoic is subdivided into three eras, from oldest to youngest they are Paleozoic (“ancient life”), Mesozoic (“middle life”), and Cenozoic (“recent life”) and the remaining three chapter headings are on these three important eras.
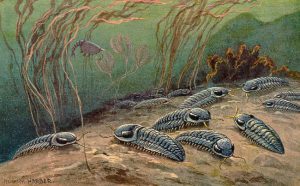
Life in the early Paleozoic Era was dominated by marine organisms but by the middle of the era plants and animals evolved to live and reproduce on land. Fish evolved jaws and fins evolved into jointed limbs. The development of lungs allowed animals to emerge from the sea and become the first air-breathing tetrapods (four-legged animals) such as amphibians. From amphibians evolved reptiles with the amniotic egg. From reptiles evolved an early ancestor to birds and mammals and their scales became feathers and fur. Near the end of the Paleozoic Era, the Carboniferous Period had some of the most extensive forests in Earth’s history. Their fossilized remains became the coal that powered the industrial revolution
8.6.1 Paleozoic Tectonics and Paleogeography
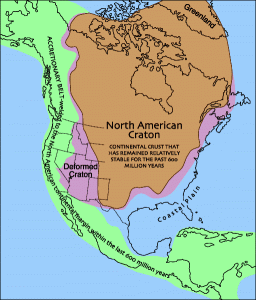
During the Paleozoic Era, sea-levels rose and fell four times. With each sea-level rise, the majority of North America was covered by a shallow tropical ocean. Evidence of these submersions are the abundant marine sedimentary rocks such as limestone with fossils corals and ooids. Extensive sea-level falls are documented by widespread unconformities. Today, the midcontinent has extensive marine sedimentary rocks from the Paleozoic and western North America has thick layers of marine limestone on block faulted mountain ranges such as Mt. Timpanogos near Provo, Utah.
The assembly of supercontinent Pangea, sometimes spelled Pangaea, was completed by the late Paleozoic Era. The name Pangea was originally coined by Alfred Wegener and means “all land.” Pangea is the when all of the major continents were grouped together as one by a series of tectonic events including subduction island-arc accretion, and continental collisions, and ocean-basin closures. In North America, these tectonic events occurred on the east coast and are known as the Taconic, Acadian, Caledonian, and Alleghanian orogenies. The Appalachian Mountains are the erosional remnants of these mountain building events in North America. Surrounding Pangea was a global ocean basin known as the Panthalassa. Continued plate movement extended the ocean into Pangea, forming a large bay called the Tethys Sea that eventually divided the land mass into two smaller supercontinents, Laurasia and Gondwana. Laurasia consisted of Laurentia and Eurasia, and Gondwana consisted of the remaining continents of South America, Africa, India, Australia, and Antarctica.
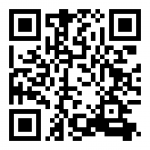
Animation of plate movement the last 3.3 billion years. Pangea occurs at the 4:40 mark.
While the east coast of North America was tectonically active during the Paleozoic Era, the west coast remained mostly inactive as a passive margin during the early Paleozoic. The western edge of North American continent was near the present-day Nevada-Utah border and was an expansive shallow continental shelf near the paleoequator. However, by the Devonian Period, the Antler orogeny started on the west coast and lasted until the Pennsylvanian Period. The Antler orogeny was a volcanic island arc that was accreted onto western North America with the subduction direction away from North America. This created a mountain range on the west coast of North American called the Antler highlands and was the first part of building the land in the west that would eventually make most of California, Oregon, and Washington states. By the late Paleozoic, the Sonoma orogeny began on the west coast and was another collision of an island arc. The Sonoma orogeny marks the change in subduction direction to be toward North America with a volcanic arc along the entire west coast of North America by late Paleozoic to early Mesozoic Eras.
By the end of the Paleozoic Era, the east coast of North America had a very high mountain range due to continental collision and the creation of Pangea. The west coast of North America had smaller and isolated volcanic highlands associated with island arc accretion. During the Mesozoic Era, the size of the mountains on either side of North America would flip, with the west coast being a more tectonically active plate boundary and the east coast changing into a passive margin after the breakup of Pangea.
8.6.2 Paleozoic Evolution
The beginning of the Paleozoic Era is marked by the first appearance of hard body parts like shells, spikes, teeth, and scales; and the appearance in the rock record of most animal phyla known today. That is, most basic animal body plans appeared in the rock record during the Cambrian Period. This sudden appearance of biological diversity is called the Cambrian Explosion. Scientists debate whether this sudden appearance is more from a rapid evolutionary diversification as a result of a warmer climate following the late Proterozoic glacial environments, better preservation and fossilization of hard parts, or artifacts of a more complete and recent rock record. For example, fauna may have been diverse during the Ediacaran Period, setting the state for the Cambrian Explosion, but they lacked hard body parts and would have left few fossils behind. Regardless, during the Cambrian Period 541–485 million years ago marked the appearance of most animal phyla.
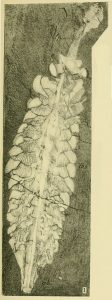
One of the best fossil sites for the Cambrian Explosion was discovered in 1909 by Charles Walcott (1850–1927) in the Burgess Shale in western Canada. The Burgess Shale is a Lagerstätte, a site of exceptional fossil preservation that includes impressions of soft body parts. This discovery allowed scientists to study Cambrian animals in immense detail because soft body parts are not normally preserved and fossilized. Other Lagerstätte sites of similar age in China and Utah have allowed scientist to form a detailed picture of Cambrian biodiversity. The biggest mystery surrounds animals that do not fit existing lineages and are unique to that time. This includes many famous fossilized creatures: the first compound-eyed trilobites; Wiwaxia, a creature covered in spiny plates; Hallucigenia, a walking worm with spikes; Opabinia, a five-eyed arthropod with a grappling claw; and Anomalocaris, the alpha predator of its time, complete with grasping appendages and circular mouth with sharp plates. Most notably appearing during the Cambrian is an important ancestor to humans. A segmented worm called Pikaia is thought to be the earliest ancestor of the Chordata phylum that includes vertebrates, animals with backbones.
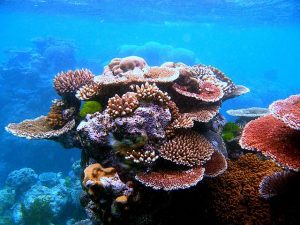
By the end of the Cambrian, mollusks, brachiopods, nautiloids, gastropods, graptolites, echinoderms, and trilobites covered the sea floor. Although most animal phyla appeared by the Cambrian, the biodiversity at the family, genus, and species level was low until the Ordovician Period. During the Great Ordovician Biodiversification Event, vertebrates and invertebrates (animals without backbone) became more diverse and complex at family, genus, and species level. The cause of the rapid speciation event is still debated but some likely causes are a combination of warm temperatures, expansive continental shelves near the equator, and more volcanism along the mid-ocean ridges. Some have shown evidence that an asteroid breakup event and consequent heavy meteorite impacts correlate with this diversification event. The additional volcanism added nutrients to ocean water helping support a robust ecosystem. Many life forms and ecosystems that would be recognizable in current times appeared at this time. Mollusks, corals, and arthropods in particular multiplied to dominate the oceans.
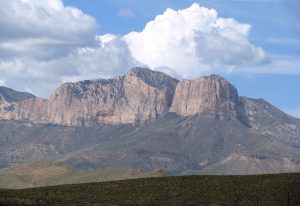
One important evolutionary advancement during the Ordovician Period was reef-building organisms, mostly colonial coral. Corals took advantage of the ocean chemistry, using calcite to build large structures that resembled modern reefs like the Great Barrier Reef off the coast of Australia. These reefs housed thriving ecosystems of organisms that swam around, hid in, and crawled over them. Reefs are important to paleontologists because of their preservation potential, massive size, and in-place ecosystems. Few other fossils offer more diversity and complexity than reef assemblages.
According to evidence from glacial deposits, a small ice age caused sea-levels to drop and led to a major mass extinction by the end of the Ordovician. This is the earliest of five mass extinction events documented in the fossil record. During this mass extinction, an unusually large number of species abruptly disappear in the fossil record (see video).
Life bounced back during the Silurian period. The major evolutionary event was the development of the forward pair of gill arches into jaws, allowing fish new feeding strategies and opening up new ecological niches.
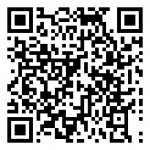
3-minute video describing mass extinctions and how they are defined.
Life bounced back during the Silurian period. The period’s major evolutionary event was the development of jaws from the forward pair of gill arches in bony fishes and sharks. Hinged jaws allowed fish to exploit new food sources and ecological niches. This period also included the start of armored fishes, known as the placoderms. In addition to fish and jaws, Silurian rocks provide the first evidence of terrestrial or land-dwelling plants and animals. The first vascular plant, Cooksonia, had woody tissues, pores for gas exchange, and veins for water and food transport. Insects, spiders, scorpions, and crustaceans began to inhabit moist, freshwater terrestrial environments.
The Devonian Period is called the Age of Fishes due to the rise in plated, jawed, and lobe-finned fishes . The lobe-finned fishes, which were related to the modern lungfish and coelacanth, are important for their eventual evolution into tetrapods, four-limbed vertebrate animals that can walk on land. The first lobe-finned land-walking fish, named Tiktaalik, appeared about 385 million years ago and serves as a transition fossil between fish and early tetrapods. Though Tiktaalik was clearly a fish, it had some tetrapod structures as well. Several fossils from the Devonian are more tetrapod like than fish like but these weren’t fully terrestrial. The first fully terrestrial tetrapod arrived in the Mississippian (early Carboniferous) period. By the Mississippian (early Carboniferous) period, tetrapods had evolved into two main groups, amphibians and amniotes, from a common tetrapod ancestor. The amphibians were able to breathe air and live on land but still needed water to nurture their soft eggs. The first reptile (an amniote) could live and reproduce entirely on land with hard-shelled eggs that wouldn’t dry out.
Land plants had also evolved into the first trees and forests. Toward the end of the Devonian, another mass extinction event occurred. This extinction, while severe, is the least temporally defined, with wide variations in the timing of the event or events. Reef building organisms were the hardest hit, leading to dramatic changes in marine ecosystems.
The next time period, called the Carboniferous (North American geologists have subdivided this into the Mississippian and Pennsylvanian periods), saw the highest levels of oxygen ever known, with forests (e.g., ferns, club mosses) and swamps dominating the landscape . This helped cause the largest arthropods ever, like the millipede Arthropleura, at 2.5 meters (6.4 feet) long! It also saw the rise of a new group of animals, the reptiles. The evolutionary advantage that reptiles have over amphibians is the amniote egg (egg with a protective shell), which allows them to rely on non-aquatic environments for reproduction. This widened the terrestrial reach of reptiles compared to amphibians. This booming life, especially plant life, created cooling temperatures as carbon dioxide was removed from the atmosphere. By the middle Carboniferous, these cooler temperatures led to an ice age (called the Karoo Glaciation) and less-productive forests. The reptiles fared much better than the amphibians, leading to their diversification. This glacial event lasted into the early Permian.
By the Permian, with Pangea assembled, the supercontinent led to a dryer climate, and even more diversification and domination by the reptiles. The groups that developed in this warm climate eventually radiated into dinosaurs. Another group, known as the synapsids, eventually evolved into mammals. Synapsids, including the famous sail-backed Dimetrodon are commonly confused with dinosaurs. Pelycosaurs (of the Pennsylvanian to early Permian like Dimetrodon) are the first group of synapsids that exhibit the beginnings of mammalian characteristics such as well-differentiated dentition: incisors, highly developed canines in lower and upper jaws and cheek teeth, premolars and molars. Starting in the late Permian, a second group of synapsids, called the therapsids (or mammal-like reptiles) evolve, and become the ancestors to mammals.
Permian Mass Extinction
The end of the Paleozoic era is marked by the largest mass extinction in earth history. The Paleozoic era had two smaller mass extinctions, but these were not as large as the Permian Mass Extinction, also known as the Permian-Triassic Extinction Event. It is estimated that up to 96% of marine species and 70% of land-dwelling (terrestrial) vertebrates went extinct. Many famous organisms, like sea scorpions and trilobites, were never seen again in the fossil record. What caused such a widespread extinction event? The exact cause is still debated, though the leading idea relates to extensive volcanism associated with the Siberian Traps, which are one of the largest deposits of flood basalts known on Earth, dating to the time of the extinction event. The eruption size is estimated at over 3 million cubic kilometers that is approximately 4,000,000 times larger than the famous 1980 Mt. St. Helens eruption in Washington. The unusually large volcanic eruption would have contributed a large amount of toxic gases, aerosols, and greenhouse gasses into the atmosphere. Further, some evidence suggests that the volcanism burned vast coal deposits releasing methane (a greenhouse gas) into the atmosphere. As discussed in Chapter 15, greenhouse gases cause the climate to warm. This extensive addition of greenhouse gases from the Siberian Traps may have caused a runaway greenhouse effect that rapidly changed the climate, acidified the oceans, disrupted food chains, disrupted carbon cycling, and caused the largest mass extinction.
Take this quiz to check your comprehension of this section.
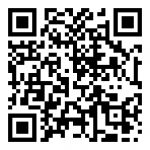
8.7 Phanerozoic Eon: Mesozoic Era
Following the Permian Mass Extinction, the Mesozoic (« middle life ») was from 252 million years ago to 66 million years ago. As Pangea started to break apart, mammals, birds, and flowering plants developed. The Mesozoic is probably best known as the age of reptiles, most notably, the dinosaurs.
8.7.1 Mesozoic Tectonics and Paleogeography
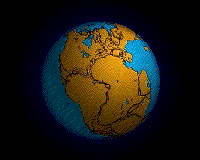
Pangea started breaking up (in a region that would become eastern Canada and United States) around 210 million years ago in the Late Triassic. Clear evidence for this includes the age of the sediments in the Newark Supergroup rift basins and the Palisades sill of the eastern part of North America and the age of the Atlantic ocean floor. Due to sea-floor spreading, the oldest rocks on the Atlantic’s floor are along the coast of northern Africa and the east coast of North America, while the youngest are along the mid-ocean ridge.
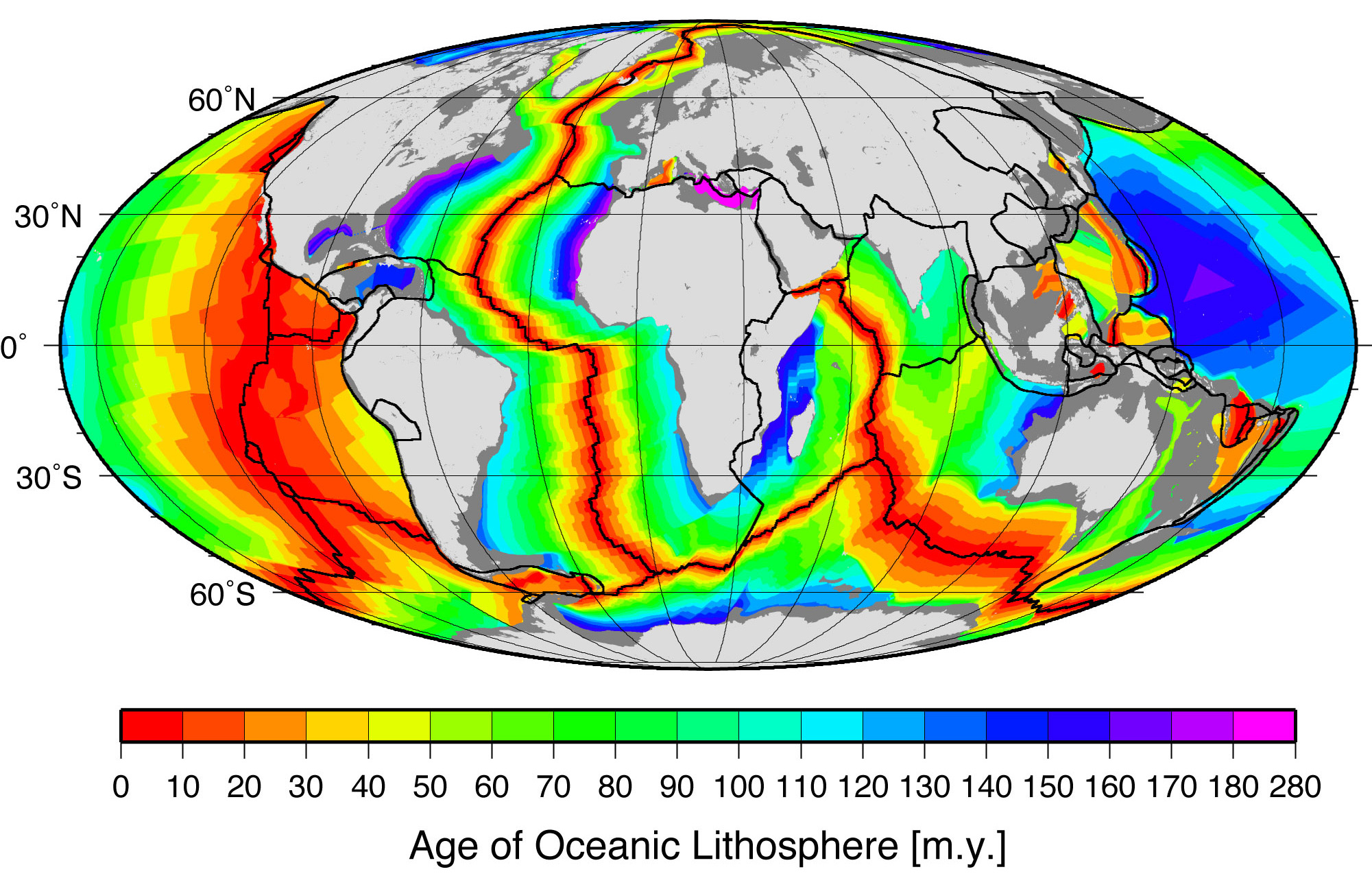
This age pattern shows how the Atlantic Ocean opened as the young Mid-Atlantic Ridge began to create the seafloor. This means the Atlantic ocean started opening and was first formed here. The southern Atlantic opened next, with South America separating from central and southern Africa. Last (happening after the Mesozoic ended) was the northernmost Atlantic, with Greenland and Scandinavia parting ways. The breaking points of each rifted plate margin eventually turned into the passive plate boundaries of the east coast of the Americas today.
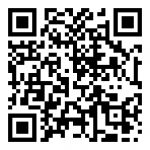
Video of Pangea breaking apart and plates moving to their present locations. By Tanya Atwater.
In western North America, an active plate margin had started with subduction, controlling most of the tectonics of that region in the Mesozoic. Another possible island-arc collision created the Sonoman Orogeny in Nevada during the latest Paleozoic to the Triassic. In the Jurassic, another island-arc collision caused the Nevadan Orogeny, a large Andean-style volcanic arc and thrust belt. The Sevier Orogeny followed in the Cretaceous, which was mainly a volcanic arc to the west and a thin-skinned fold and thrust belt to the east, meaning stacks of shallow faults and folds built up the topography. Many of the structures in the Rocky Mountains today date from this orogeny.
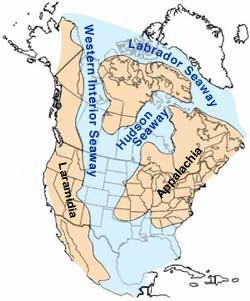
Tectonics had an influence in one more important geographic feature in North America: the Cretaceous Western Interior Foreland Basin, which flooded during high sea levels forming the Cretaceous Interior Seaway. Subduction from the west was the Farallon Plate, an oceanic plate connected to the Pacific Plate (seen today as remnants such as the Juan de Fuca Plate, off the coast of the Pacific Northwest). Subduction was shallow at this time because a very young, hot and less dense portion of the Farallon plate was subducted. This shallow subduction caused a downwarping in the central part of North America. High sea levels due to shallow subduction, and increasing rates of seafloor spreading and subduction, high temperatures, and melted ice also contributed to the high sea levels. These factors allowed a shallow epicontinental seaway that extended from the Gulf of Mexico to the Arctic Ocean to divide North America into two separate land masses, Laramidia to the west and Appalachia to the east, for 25 million years. Many of the coal deposits in Utah and Wyoming formed from swamps along the shores of this seaway. By the end of the Cretaceous, cooling temperatures caused the seaway to regress.
8.7.2 Mesozoic Evolution
The Mesozoic era is dominated by reptiles, and more specifically, the dinosaurs. The Triassic saw devastated ecosystems that took over 30 million years to fully re-emerge after the Permian Mass Extinction. The first appearance of many modern groups of animals that would later flourish occurred at this time. This includes frogs (amphibians), turtles (reptiles), marine ichthyosaurs and plesiosaurs (marine reptiles), mammals, and the archosaurs. The archosaurs (“ruling reptiles”) include ancestral groups that went extinct at the end of the Triassic, as well as the flying pterosaurs, crocodilians, and the dinosaurs. Archosaurs, like the placental mammals after them, occupied all major environments: terrestrial (dinosaurs), in the air (pterosaurs), aquatic (crocodilians) and even fully marine habitats (marine crocodiles). The pterosaurs, the first vertebrate group to take flight, like the dinosaurs and mammals, start small in the Triassic.
At the end of the Triassic, another mass extinction event occurred, the fourth major mass extinction in the geologic record. This was perhaps caused by the Central Atlantic Magmatic Province flood basalt. The end-Triassic extinction made certain lineages go extinct and helped spur the evolution of survivors like mammals, pterosaurs (flying reptiles), ichthyosaurs/plesiosaurs/mosasaurs (marine reptiles), and dinosaurs.
Mammals, as previously mentioned, got their start from a reptilian synapsid ancestor possibly in the late Paleozoic. Mammals stayed small, in mainly nocturnal niches, with insects being their largest prey. The development of warm-blooded circulation and fur may have been a response to this lifestyle.
In the Jurassic, species that were previously common, flourished due to a warmer and more tropical climate. The dinosaurs were relatively small animals in the Triassic period of the Mesozoic, but became truly massive in the Jurassic. Dinosaurs are split into two groups based on their hip structure, i.e. orientation of the pubis and ischium bones in relationship to each other. This is referred to as the “reptile hipped” saurischians and the “bird hipped” ornithischians. This has recently been brought into question by a new idea for dinosaur lineage.
Most of the dinosaurs of the Triassic were saurischians, but all of them were bipedal. The major adaptive advantage dinosaurs had was changes in the hip and ankle bones, tucking the legs under the body for improved locomotion as opposed to the semi-erect gait of crocodiles or the sprawling posture of reptiles. In the Jurassic, limbs (or a lack thereof) were also important to another group of reptiles, leading to the evolution of Eophis, the oldest snake.
There is a paucity of dinosaur fossils from the Early and Middle Jurassic, but by the Late Jurassic they were dominating the planet. The saurischians diversified into the giant herbivorous (plant-eating) long-necked sauropods weighing up to 100 tons and bipedal carnivorous theropods, with the possible exception of the Therizinosaurs. All of the ornithischians (e.g Stegosaurus, Iguanodon, Triceratops, Ankylosaurus, Pachycephhlosaurus) were herbivorous with a strong tendency to have a “turtle-like” beak at the tips of their mouths.
The pterosaurs grew and diversified in the Jurassic, and another notable arial organism developed and thrived in the Jurassic: birds. When Archeopteryx was found in the Solnhofen Lagerstätte of Germany, a seeming dinosaur-bird hybrid, it started the conversation on the origin of birds. The idea that birds evolved from dinosaurs occurred very early in the history of research into evolution, only a few years after Darwin’s On the Origin of Species. This study used a remarkable fossil of Archeopteryx from a transitional animal between dinosaurs and birds. Small meat-eating theropod dinosaurs were likely the branch that became birds due to their similar features. A significant debate still exists over how and when powered flight evolved. Some have stated a running-start model, while others have favored a tree-leaping gliding model or even a semi-combination: flapping to aid in climbing.
The Cretaceous saw a further diversification, specialization, and domination of the dinosaurs and other fauna. One of the biggest changes on land was the transition to angiosperm-dominated flora. Angiosperms, which are plants with flowers and seeds, had originated in the Cretaceous, switching many plains to grasslands by the end of the Mesozoic. By the end of the period, they had replaced gymnosperms (evergreen trees) and ferns as the dominant plant in the world’s forests. Haplodiploid eusocial insects (bees and ants) are descendants from Jurassic wasp-like ancestors that co-evolved with the flowering plants during this time period. The breakup of Pangea not only shaped our modern world’s geography, but biodiversity at the time as well. Throughout the Mesozoic, animals on the isolated, now separated island continents (formerly parts of Pangea), took strange evolutionary turns. This includes giant titanosaurian sauropods (Argentinosaurus) and theropods (Giganotosaurus) from South America.
K-T Extinction
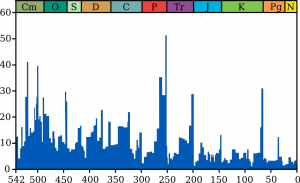
Similar to the end of the Paleozoic era, the Mesozoic Era ended with the K-Pg Mass Extinction (previously known as the K-T Extinction) 66 million years ago. This extinction event was likely caused by a large bolide (an extraterrestrial impactor such as an asteroid, meteoroid, or comet) that collided with earth. Ninety percent of plankton species, 75% of plant species, and all the dinosaurs went extinct at this time.
One of the strongest pieces of evidence comes from the element iridium. Quite rare on Earth, and more common in meteorites, it has been found all over the world in higher concentrations at a particular layer of rock that formed at the time of the K-T boundary. Soon other scientists started to find evidence to back up the claim. Melted rock spheres, a special type of “shocked” quartz called stishovite, that only is found at impact sites, was found in many places around the world . The huge impact created a strong thermal pulse that could be responsible for global forest fires, strong acid rains, a corresponding abundance of ferns, the first colonizing plants after a forest fire, enough debris thrown into the air to significantly cool temperatures afterward, and a 2-km high tsunami inferred from deposits found from Texas to Alabama.
Still, with all this evidence, one large piece remained missing: the crater where the bolide impacted. It was not until 1991 that the crater was confirmed using petroleum company geophysical data. Even though it is the third largest confirmed crater on Earth at roughly 180 km wide, the Chicxulub Crater was hard to find due to being partially underwater and partially obscured by the dense forest canopy of the Yucatan Peninsula. Coring of the center of the impact called the peak ring contained granite, indicating the impact was so powerful that it lifted basement sediment from the crust several miles toward the surface. In 2010, an international team of scientists reviewed 20 years of research and blamed the impact for the extinction.
With all of this information, it seems like the case would be closed. However, there are other events at this time which could have partially aided the demise of so many organisms. For example, sea levels are known to be slowly decreasing at the time of the K-T event, which is tied to marine extinctions, though any study on gradual vs. sudden changes in the fossil record is flawed due to the incomplete nature of the fossil record. Another big event at this time was the Deccan Traps flood basalt volcanism in India. At over 1.3 million cubic kilometers of material, it was certainly a large source of material hazardous to ecosystems at the time, and it has been suggested as at least partially responsible for the extinction. Some have found the impact and eruptions too much of a coincidence, and have even linked the two together.
Take this quiz to check your comprehension of this section.
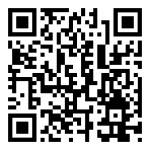
8.8 Phanerozoic Eon: Cenozoic Era
The Cenozoic, meaning “new life,” is known as the age of mammals because it is in this era that mammals came to be a dominant and large life form, including human ancestors. Birds, as well, flourished in the open niches left by the dinosaur’s demise. Most of the Cenozoic has been relatively warm, with the main exception being the ice age that started about 2.558 million years ago and (despite recent warming) continues today. Tectonic shifts in the west caused volcanism, but eventually changed the long-standing subduction zone into a transform boundary.
8.8.1 Cenozoic Tectonics and Paleogeography
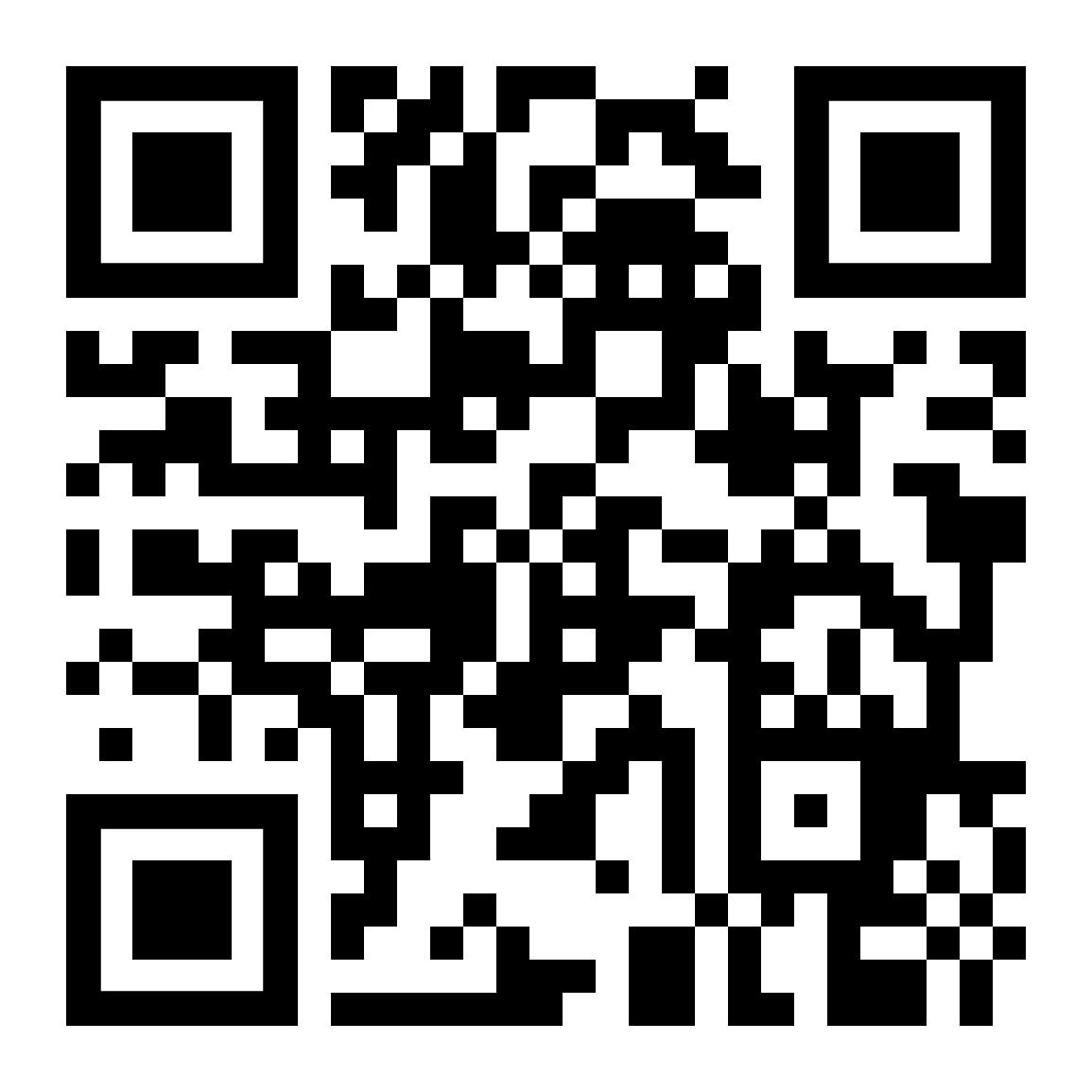
Animation of the last 38 million years of movement in western North America. Note, that after the ridge is subducted, convergent turns to transform (with divergent inland).
In the Cenozoic, the plates of the Earth moved into more familiar places, with the biggest change being the closing of the Tethys Sea with collisions such as the Alps, Zagros, and Himalaya, a collision that started about 57 million years ago, and continues today. Maybe the most significant tectonic feature that occurred in the Cenozoic of North America was the conversion of the west coast of California from a convergent boundary subduction zone to a transform boundary. Subduction off the coast of the western United States, which had occurred throughout the Mesozoic, had continued in the Cenozoic. After the Sevier Orogeny in the late Mesozoic, a subsequent orogeny called the Laramide Orogeny, occurred in the early Cenozoic. The Laramide was thick-skinned, different than the Sevier Orogeny. It involved deeper crustal rocks, and produced bulges that would become mountain ranges like the Rockies, Black Hills, Wind River Range, Uinta Mountains, and the San Rafael Swell. Instead of descending directly into the mantle, the subducting plate shallowed out and moved eastward beneath the continental plate affecting the overlying continent hundreds of miles east of the continental margin and building high mountains. This occurred because the subducting plate was so young and near the spreading center and the density of the plate was therefore low and subduction was hindered.
As the mid-ocean ridge itself started to subduct, the relative motion had changed. Subduction caused a relative convergence between the subducting Farallon plate and the North American plate. On the other side of the mid-ocean ridge from the Farallon plate was the Pacific plate, which was moving away from the North American plate. Thus, as the subduction zone consumed the mid-ocean ridge, the relative movement became transform instead of convergent, which went on to become the San Andreas Fault System. As the San Andreas grew, it caused east-west directed extensional forces to spread over the western United States, creating the Basin and Range province. The transform fault switched position over the last 18 million years, twisting the mountains around Los Angeles, and new faults in the southeastern California deserts may become a future San Andreas-style fault. During this switch from subduction to transform, the nearly horizontal Farallon slab began to sink into the mantle. This caused magmatism as the subducting slab sank, allowing asthenosphere material to rise around it. This event is called the Oligocene ignimbrite flare-up, which was one of the most significant periods of volcanism ever, including the largest single confirmed eruption, the 5000 cubic kilometer Fish Canyon Tuff.
8.8.2 Cenozoic Evolution
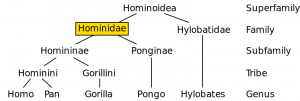
There are five groups of early mammals in the fossil record, based primarily on fossil teeth, the hardest bone in vertebrate skeletons. For the purpose of this text, the most important group are the Eupantotheres, that diverge into the two main groups of mammals, the marsupials (like Sinodelphys) and placentals or eutherians (like Eomaia) in the Cretaceous and then diversified in the Cenozoic. The marsupials dominated on the isolated island continents of South America and Australia, and many went extinct in South America with the introduction of placental mammals. Some well-known mammal groups have been highly studied with interesting evolutionary stories in the Cenozoic. For example, horses started small with four toes, ended up larger and having just one toe. Cetaceans (marine mammals like whales and dolphins) started on land from small bear-like (mesonychids) creatures in the early Cenozoic and gradually took to water. However, no study of evolution has been more studied than human evolution. Hominids, the name for human-like primates, started in eastern Africa several million years ago.
The first critical event in this story is an environmental change from jungle to more of a savanna, probably caused by changes in Indian Ocean circulation. While bipedalism is known to have evolved before this shift, it is generally believed that our bipedal ancestors (like Australopithecus) had an advantage by covering ground more easily in a more open environment compared to their non-bipedal evolutionary cousins. There is also a growing body of evidence, including the famous “Lucy” fossil of an Australopithecine, that our early ancestors lived in trees. Arboreal animals usually demand a high intelligence to navigate through a three-dimensional world. It is from this lineage that humans evolved, using endurance running as a means to acquire more resources and possibly even hunt. This can explain many uniquely human features, from our long legs, strong achilles, lack of lower gut protection, and our wide range of running efficiencies.
Now that the hands are freed up, the next big step is a large brain. There have been arguments from a switch to more meat eating, cooking with fire, tool use, and even the construct of society itself to explain this increase in brain size. Regardless of how, it was this increased cognitive power that allowed humans to reign as their ancestors moved out of Africa and explored the world, ultimately entering the Americas through land bridges like the Bering Land Bridge. The details of this worldwide migration and the different branches of the hominid evolutionary tree are very complex, and best reserved for its own course.
Anthropocene and Extinction
Humans have had an influence on the Earth, its ecosystems and climate. Yet, human activity can not explain all of the changes that have occurred in the recent past. The start of the Quaternary period, the last and current period of the Cenozoic, is marked by the start of our current ice age 2.58 million years ago. During this time period, ice sheets advanced and retreated, most likely due to Milankovitch cycles (see ch. 15). Also at this time, various cold-adapted megafauna emerged (like giant sloths, saber-tooth cats, and woolly mammoths), and most of them went extinct as the Earth warmed from the most recent glacial maximum. A long-standing debate is over the cause of these and other extinctions. Is climate warming to blame, or were they caused by humans? Certainly, we know of recent human extinctions of animals like the dodo or passenger pigeon. Can we connect modern extinctions to extinctions in the recent past? If so, there are several ideas as to how this happened. Possibly the most widely accepted and oldest is the hunting/overkill hypothesis. The idea behind this hypothesis is that humans hunted large herbivores for food, then carnivores could not find food, and human arrival times in locations has been shown to be tied to increased extinction rates in many cases.
Modern human impact on the environment and the Earth as a whole is unquestioned. In fact, many scientists are starting to suggest that the rise of human civilization ended and/or replaced the Holocene epoch and defines a new geologic time interval: the Anthropocene. Evidence for this change includes extinctions, increased tritium (hydrogen with two neutrons) due to nuclear testing, rising pollutants like carbon dioxide, more than 200 never-before seen mineral species that have occurred only in this epoch, materials such as plastic and metals which will be long lasting « fossils » in the geologic record, and large amounts of earthen material moved. The biggest scientific debate with this topic is the starting point. Some say that humans’ invention of agriculture would be recognized in geologic strata and that should be the starting point, around 12,000 years ago. Others link the start of the industrial revolution and the subsequent addition of vast amounts of carbon dioxide in the atmosphere. Either way, the idea is that alien geologists visiting Earth in the distant future would easily recognize the impact of humans on the Earth as the beginning of a new geologic period.
Take this quiz to check your comprehension of this section.
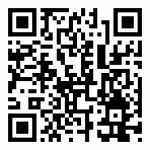
Summary
The changes that have occurred since the inception of Earth are vast and significant. From the oxygenation of the atmosphere, the progression of life forms, the assembly and deconstruction of several supercontinents, to the extinction of more life forms than exist today, having a general understanding of these changes can put present change into a more rounded perspective.
Take this quiz to check your comprehension of this Chapter.
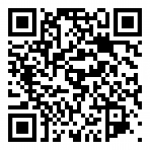
References
- Alvarez, L.W., Alvarez, W., Asaro, F., and Michel, H.V., 1980, Extraterrestrial cause for the cretaceous-tertiary extinction: Science, v. 208, no. 4448, p. 1095–1108.
- Beerling, D., 2008, The emerald planet: how plants changed Earth’s history: OUP Oxford.
- Boyce, J.W., Liu, Y., Rossman, G.R., Guan, Y., Eiler, J.M., Stolper, E.M., and Taylor, L.A., 2010, Lunar apatite with terrestrial volatile abundances: Nature, v. 466, no. 7305, p. 466–469.
- Brueckner, H.K., and Snyder, W.S., 1985, Structure of the Havallah sequence, Golconda allochthon, Nevada: Evidence for prolonged evolution in an accretionary prism: Geol. Soc. Am. Bull., v. 96, no. 9, p. 1113–1130.
- Brusatte, S.L., Benton, M.J., Ruta, M., and Lloyd, G.T., 2008, The first 50 Myr of dinosaur evolution: macroevolutionary pattern and morphological disparity: Biol. Lett., v. 4, no. 6, p. 733–736.
- Canup, R.M., and Asphaug, E., 2001, Origin of the Moon in a giant impact near the end of the Earth’s formation: Nature, v. 412, no. 6848, p. 708–712.
- Clack, J.A., 2009, The Fish–Tetrapod Transition: New Fossils and Interpretations: Evolution: Education and Outreach, v. 2, no. 2, p. 213–223., doi: 10/cz257q.
- Cohen, K.M., Finney, S.C., Gibbard, P.L., and Fan, J.-X., 2013, The ICS International Chronostratigraphic Chart: Episodes, v. 36, no. 3, p. 199–204.
- Colbert, E.H., and Morales, M.A., 1991, History of the Backboned Animals Through Time: New York: Wiley.
- De Laubenfels, M.W., 1956, Dinosaur extinction: one more hypothesis: J. Paleontol.
- Gomes, R., Levison, H.F., Tsiganis, K., and Morbidelli, A., 2005, Origin of the cataclysmic Late Heavy Bombardment period of the terrestrial planets: Nature, v. 435, no. 7041, p. 466–469.
- Hatcher, R.D., Jr, Thomas, W.A., and Viele, G.W., 1989, The Appalachian-Ouachita Orogen in the United States: Geological Society of America.
- Hosono, N., Karato, S., Makino, J., and Saitoh, T.R., 2019, Terrestrial magma ocean origin of the Moon: Nature Geoscience, p. 1., doi: 10.1038/s41561-019-0354-2.
- Hsiao, E., 2004, Possibility of life on Europa:
- Hubble, E., 1929, A relation between distance and radial velocity among extra-galactic nebulae: Proc. Natl. Acad. Sci. U. S. A., v. 15, no. 3, p. 168–173.
- Ingersoll, R.V., 1982, Triple-junction instability as cause for late Cenozoic extension and fragmentation of the western United States: Geology, v. 10, no. 12, p. 621–624.
- Johnson, C.M., 1991, Large-scale crust formation and lithosphere modification beneath Middle to Late Cenozoic calderas and volcanic fields, western North America: J. Geophys. Res. [Solid Earth], v. 96, no. B8, p. 13485–13507.
- Kass, M.S., 1999, Prognathodon stadtmani:(Mosasauridae) a new species from the Mancos Shale (lower Campanian) of western Colorado: Vertebrate Paleontology in Utah, Utah Geological.
- Livaccari, R.F., 1991, Role of crustal thickening and extensional collapse in the tectonic evolution of the Sevier-Laramide orogeny, western United States: Geology, v. 19, no. 11, p. 1104–1107.
- McMenamin, M.A., and Schulte McMenamin, D.L., 1990, The Emergence of Animals: The Cambrian Breakthrough: Columbia University Press.
- Mitrovica, J.X., Beaumont, C., and Jarvis, G.T., 1989, Tilting of continental interiors by the dynamical effects of subduction: Tectonics.
- Rücklin, M., Donoghue, P.C.J., Johanson, Z., Trinajstic, K., Marone, F., and Stampanoni, M., 2012, Development of teeth and jaws in the earliest jawed vertebrates: Nature, v. 491, no. 7426, p. 748–751.
- Sahney, S., and Benton, M.J., 2008, Recovery from the most profound mass extinction of all time: Proc. Biol. Sci., v. 275, no. 1636, p. 759–765.
- Salaris, M., and Cassisi, S., 2005, Evolution of stars and stellar populations: John Wiley & Sons.
- Schoch, R.R., 2012, Amphibian Evolution: The life of Early Land Vertebrates: Wiley-Blackwell.
- Sharp, B.J., 1958, MINERALIZATION IN THE INTRUSIVE ROCKS IN LITTLE COTTONWOOD CANYON, UTAH: GSA Bulletin, v. 69, no. 11, p. 1415–1430., doi: 10.1130/0016-7606(1958)69[1415:MITIRI]2.0.CO;2.
- Wiechert, U., Halliday, A.N., Lee, D.C., Snyder, G.A., Taylor, L.A., and Rumble, D., 2001, Oxygen isotopes and the moon-forming giant impact: Science, v. 294, no. 5541, p. 345–348.
- Wilde, S.A., Valley, J.W., Peck, W.H., and Graham, C.M., 2001, Evidence from detrital zircons for the existence of continental crust and oceans on the Earth 4.4 Gyr ago: Nature, v. 409, no. 6817, p. 175–178.
- Wood, R.A., 2019, The rise of Animals.: Scientific American, v. 320, no. 6, p. 24–31.
Mention de la source du contenu multimédia
- HubbleDeepField
- Example of Doppler Shift Youtube QR Code
- Crab_Nebula
- blackhole_NASA_2019
- 8.1 Did I Get It QR Code
- HL_Tau_protoplanetary_disk
- Artist’s impression of the water snowline around the young sta
- 8.2 Did I Get It QR Code
- NASA Evolution of the Moon Youtube QR Code
- Comet_on_7_July_2015_NavCam
- 8.3 Did I Get It QR Code
- Archean
- Pluto-in-true-color_2x_JPEG-edit-frame
- Lhborbits
- Earth cutaway schematic-en
- greenhouse-gas-molecules
- 8.4.3 Animation QR Code
- 8.4 Did I Get It QR Code
- 8.5 Did I Get It QR Code
- Trilobite_Heinrich_Harder
- North_america_craton_nps
- Opabinia
- Coral_Outcrop_Flynn_Reef
- 08.6_Guadalupe_Nima2
- How Many Mass Extinctions Youtube QR Code
- 8.7 Video QR Code
- Pangea_animation_03
- Cretaceous_seaway
- Extinction_intensity.svg
- 8.7 Did I Get It QR Code
- Hominidae_chart.svg
- 8.8 Did I Get It QR Code
- Ch.8 Review QR Code
Large surface mine with opening carved into the ground.
[glossary]
A very fine grained version of silica deposited with or without microfossils.
A chemical or biochemical rock made of mainly calcite.
Limestone made of primarily fine-grained calcite mud. Microscopic fossils are commonly present.
Extremely thin bedding in mudstones, a characteristic of shale.
Rocks which allow petroleum resources to collect or move.
Rock with abraded surfaces formed in deserts.
Discernible layers of rock, typically from a sedimentary rock.
Subtle ridges formed in the upper flow regime on top of plane beds in the direction of flow.
QR Code generated with QRCode Monkey. All generated QR Codes are 100% free and can be used for whatever you want. This includes all commercial purposes.
Lake that fills a glacial valley.
QR Code generated with QRCode Monkey. All generated QR Codes are 100% free and can be used for whatever you want. This includes all commercial purposes.
By Krishnavedala (Own work) [CC0], via Wikimedia Commons
A very fine-grained rock with very thin layering (fissile).
The process that turns non-desert land into desert.
The ability for the atmosphere to absorb heat that is emitted by a planet's surface.
Climate changed caused by human activity, namely, the burning of fossil fuels.
Having to do with humans.
An evaporite mineral, CaSo4•2H2O. Has one cleavage, hardness of 2. Typically clear or white.
Valuable material in the Earth, typically used for metallic mineral resources.
Also known as rock salt, or table salt. 3 cleavages at 90°, cubic crystal habit. Typically clear or white, hardness of 3.
A chemical sedimentary rock that forms as water evaporates.
Spheres of calcite that form in saline waters with slight wave agitation. Ooid refers to the sphere, oolite the rock with the spheres.
Porous variety of carbonate that form in relatively unheated water, sometimes as towers and spires.
Sedimentary rocks made of mineral grains weathered as mechanical detritus of previous rocks, e.g. sand, gravel, etc.
The thin, outer layer of the Earth which makes up the rocky bottom of the ocean basins. It is made of rocks similar to basalt, and as it cools, even become more dense than the upper mantle below.
Porous, concentric, or layered variety of carbonate that forms with often heated water in springs and/or caves.
A sedimentary rock that formed long ago as free oxygen changed the solubility of iron, causing layers of iron rich and iron-poor sediments to form in thin layers, or bands.
A rule that says the outer valence shell of electrons is complete when it contains 8 electrons.
Chemical sedimentary rocks that have a biologic component to their origin. Many limestones are biochemical.
Dunes that form semicircular shapes due to anchoring vegetation.
Dangerous flooding that occurs in arid regions.
Lowest layer of the soil (C), which is mechanically weathered (not chemically weathered) bedrock.
QR Code generated with QRCode Monkey. All generated QR Codes are 100% free and can be used for whatever you want. This includes all commercial purposes.
By Zkeizars (Own work) [GFDL or CC BY-SA 4.0-3.0-2.5-2.0-1.0], via Wikimedia Commons
Metallic mineral deposit consisting of mafic plutonic rocks, typically containing platinum-group elements, chromium, copper, nickel, etc.
Minerals that have a luster that is not similar to metal, and typically do not contain valuable metals like copper, lead, zinc, tin, etc.
Planar flow of water over land surfaces.
Mix of sediments that form as a subducting plate descends and the overriding plate scrapes material and material is added.
Erosional rock face caused by sand abrasion.
A process where an oceanic plate descends bellow a less dense plate, causing the removal of the plate from the surface. Subduction causes the largest earthquakes possible, as the subducting plate can lock as it goes down. Volcanism is also caused as the plate releases volatiles into the mantle, causing melting.
Name given to the subducting plate, where volatiles are driven out at depth, causing volcanism.
Potentially extractible and valuable material, but unproven.
A rock made of primarily silt.
Limestone made of shell fragments cemented together.
A dark liquid fossil fuel derived from petroleum.
Oxidation that occurs in sulfide deposits which can concentrate valuable elements like copper.
Glaciers that form in cool or mountainous areas.
A body of ice that moves downhill under its own mass.
Minerals with a luster similar to metal and contain metals, including valuable elements like lead, zinc, copper, tin, etc.
A system which reverts back to a baseline when it deviates.
A limestone made of coccolithophore shells, a type of single-celled algae.
Place where oceanic-oceanic subduction causes volcanoes to form on an overriding oceanic plate, making a chain of active volcanoes.
Former swamp-derived (plant) material that is part of the rock record.
Term for a rock made definitively of glacial till.
Metallic mineral deposit which forms near mid-ocean ridges.
Lower layer of the soil (B) which is a mixture of weathered bedrock, leeched materials, and organic material. Has two sublayers: the upper part, or regolith (with more organic materials), and the lower part, saprolite, which is only slightly weathered bedrock.
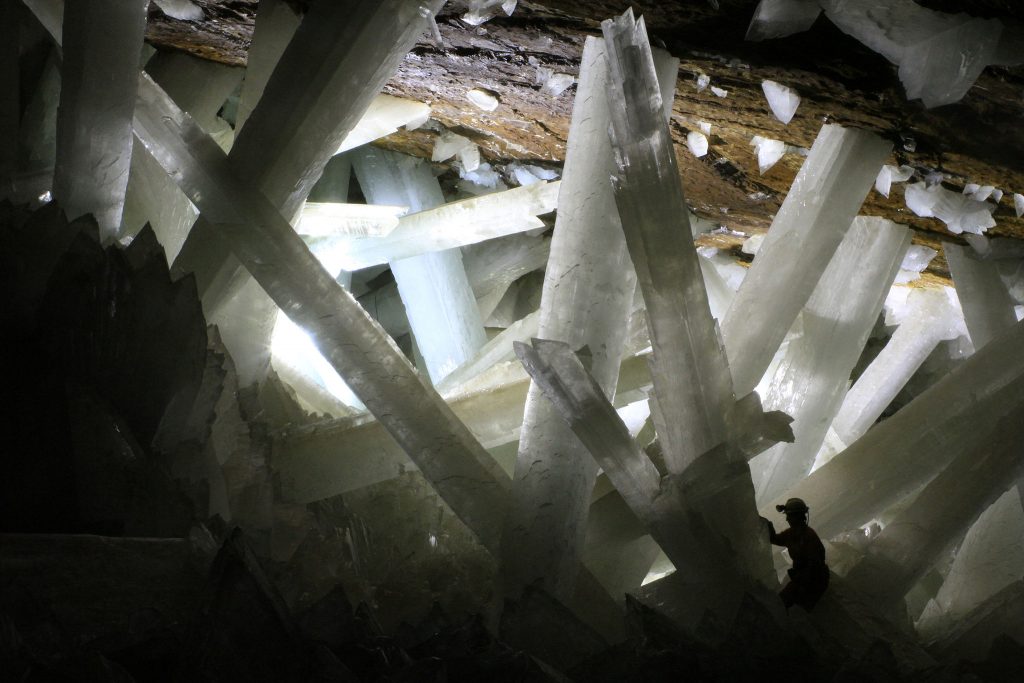
3 Minerals
KEY CONCEPTS
At the end of this chapter, students should be able to:
- Define mineral.
- Describe the basic structure of the atom.
- Derive basic atomic information from the Periodic Table of Elements.
- Describe chemical bonding related to minerals.
- Describe the main ways minerals form.
- Describe the silicon-oxygen tetrahedron and how it forms common silicate minerals.
- List common non-silicate minerals in oxide, sulfide, sulfate, and carbonate groups.
- Identify minerals using physical properties and identification tables.
The term “minerals” as used in nutrition labels and pharmaceutical products is not the same as a mineral in a geological sense. In geology, the classic definition of a mineral is: 1) naturally occurring, 2) inorganic, 3) solid at room temperature, 4) regular crystal structure, and 5) defined chemical composition. Some natural substances technically should not be considered minerals, but are included by exception. For example, water and mercury are liquid at room temperature. Both are considered minerals because they were classified before the room-temperature rule was accepted as part of the definition. Calcite is quite often formed by organic processes, but is considered a mineral because it is widely found and geologically important. Because of these discrepancies, the International Mineralogical Association in 1985 amended the definition to: “A mineral is an element or chemical compound that is normally crystalline and that has been formed as a result of geological processes.” This means that the calcite in the shell of a clam is not considered a mineral. But once that clam shell undergoes burial, diagenesis, or other geological processes, then the calcite is considered a mineral. Typically, substances like coal, pearl, opal, or obsidian that do not fit the definition of mineral are called mineraloids.
A rock is a substance that contains one or more minerals or mineraloids. As is discussed in later chapters, there are three types of rocks composed of minerals: igneous (rocks crystallizing from molten material), sedimentary (rocks composed of products of mechanical weathering (sand, gravel, etc.) and chemical weathering (things precipitated from solution), and metamorphic (rocks produced by alteration of other rocks by heat and pressure.
3.1 Chemistry of Minerals
Rocks are composed of minerals that have a specific chemical composition. To understand mineral chemistry, it is essential to examine the fundamental unit of all matter, the atom.
3.1.1 The Atom
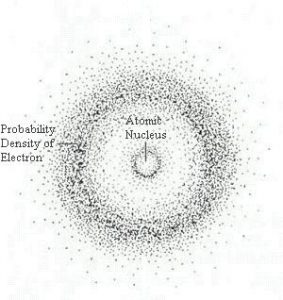
Matter is made of atoms. Atoms consists of subatomic particles—protons, neutrons, and electrons. A simple model of the atom has a central nucleus composed of protons, which have positive charges, and neutrons which have no charge. A cloud of negatively charged electrons surrounds the nucleus, the number of electrons equaling the number of protons thus balancing the positive charge of the protons for a neutral atom. Protons and neutrons each have a mass number of 1. The mass of an electron is less than 1/1000th that of a proton or neutron, meaning most of the atom’s mass is in the nucleus.
3.1.2 Periodic Table of the Elements
Matter is composed of elements which are atoms that have a specific number of protons in the nucleus. This number of protons is called the Atomic Number for the element. For example, an oxygen atom has 8 protons and an iron atom has 26 protons. An element cannot be broken down chemically into a simpler form and retains unique chemical and physical properties. Each element behaves in a unique manner in nature. This uniqueness led scientists to develop a periodic table of the elements, a tabular arrangement of all known elements listed in order of their atomic number.
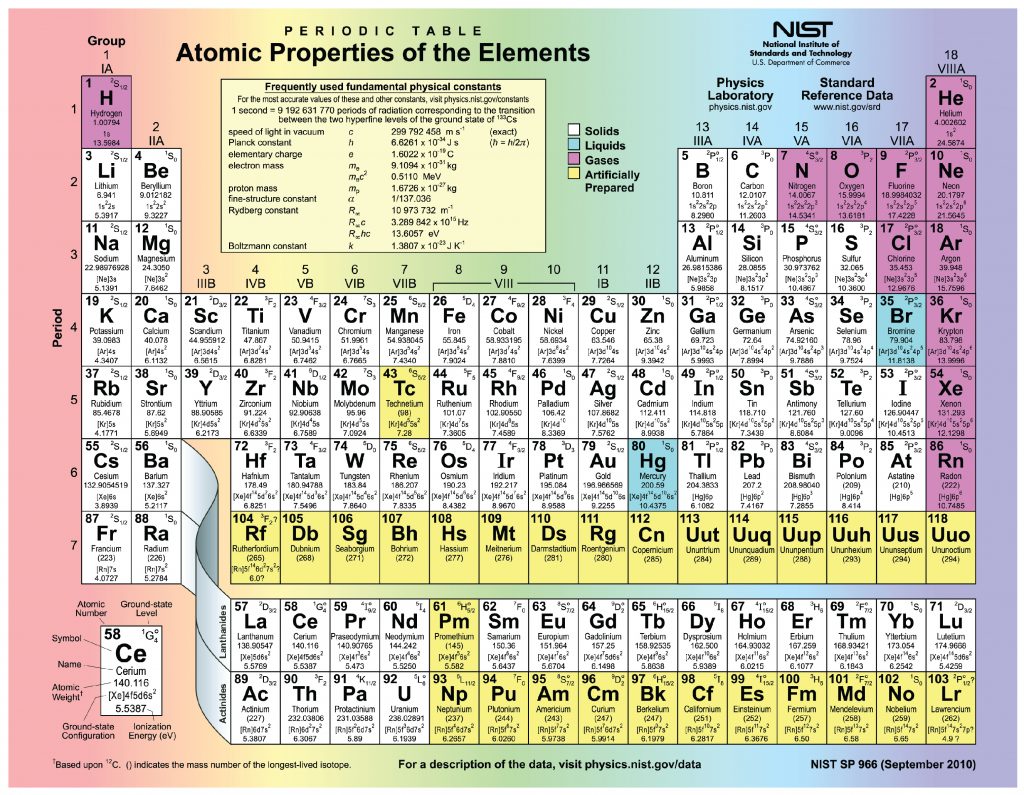
The first arrangement of elements into a periodic table was done by Dmitri Mendeleev in 1869 using the elements known at the time. In the periodic table, each element has a chemical symbol, name, atomic number, and atomic mass. The chemical symbol is an abbreviation for the element, often derived from a Latin or Greek name for the substance. The atomic number is the number of protons in the nucleus. The atomic mass is the number of protons and neutrons in the nucleus, each with a mass number of one. Since the mass of electrons is so much less than the protons and neutrons, the atomic mass is effectively the number of protons plus neutrons.
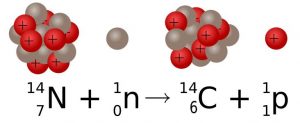
The atomic mass of natural elements represents an average mass of the atoms comprising that substance in nature and is usually not a whole number as seen on the periodic table, meaning that an element exists in nature with atoms having different numbers of neutrons. The differing number of neutrons affects the mass of an element in nature and the atomic mass number represents this average. This gives rise to the concept of isotope. Isotopes are forms of an element with the same number of protons but different numbers of neutrons. There are usually several isotopes for a particular element. For example, 98.9% of carbon atoms have 6 protons and 6 neutrons. This isotope of carbon is called carbon-12 (12C). A few carbon atoms, carbon-13 (13C), have 6 protons and 7 neutrons. A trace amount of carbon atoms, carbon-14 (14C), has 6 protons and 8 neutrons.
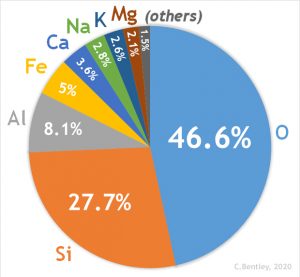
Among the 118 known elements, the heaviest are fleeting human creations known only in high energy particle accelerators, and they decay rapidly. The heaviest naturally occurring element is uranium, atomic number 92. The eight most abundant elements in Earth’s continental crust are shown in Table 1. These elements are found in the most common rock forming minerals.
Element | Symbol | Abundance % |
Oxygen | O | 47% |
Silicon | Si | 28% |
Aluminum | Al | 8% |
Iron | Fe | 5% |
Calcium | Ca | 4% |
Sodium | Na | 3% |
Potassium | K | 3% |
Magnesium | Mg | 2% |
Table 1. Eight Most Abundant Elements in the Earth’s Continental Crust % by weight (source: USGS). All other elements are less than 1%.
3.1.3 Chemical Bonding
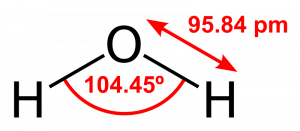
Most substances on Earth are compounds containing multiple elements. Chemical bonding describes how these atoms attach with each other to form compounds, such as sodium and chlorine combining to form NaCl, common table salt. Compounds that are held together by chemical bonds are called molecules. Water is a compound of hydrogen and oxygen in which two hydrogen atoms are covalently bonded with one oxygen making the water molecule. The oxygen we breathe is formed when one oxygen atom covalently bonds with another oxygen atom to make the molecule O2. The subscript 2 in the chemical formula indicates the molecule contains two atoms of oxygen.
Most minerals are also compounds of more than one element. The common mineral calcite has the chemical formula CaCO3 indicating the molecule consists of one calcium, one carbon, and three oxygen atoms. In calcite, one carbon and three oxygen atoms are held together by covalent bonds to form a molecular ion, called carbonate, which has a negative charge. Calcium as an ion has a positive charge of plus two. The two oppositely charged ions attract each other and combine to form the mineral calcite, CaCO3. The name of the chemical compound is calcium carbonate, where calcium is Ca and carbonate refers to the molecular ion CO3-2.
The mineral olivine has the chemical formula (Mg,Fe)2SiO4, in which one silicon and four oxygen atoms are bonded with two atoms of either magnesium or iron. The comma between iron (Fe) and magnesium (Mg) indicates the two elements can occupy the same location in the crystal structure and substitute for one another.
3.1.3.1 Valence and Charge
The electrons around the atom’s nucleus are located in shells representing different energy levels. The outermost shell is called the valence shell. Electrons in the valence shell are involved in chemical bonding. In 1913, Niels Bohr proposed a simple model of the atom that states atoms are more stable when their outermost shell is full. Atoms of most elements thus tend to gain or lose electrons so the outermost or valence shell is full. In Bohr’s model, the innermost shell can have a maximum of two electrons and the second and third shells can have a maximum of eight electrons. When the innermost shell is the valence shell, as in the case of hydrogen and helium, it obeys the octet rule when it is full with two electrons. For elements in higher rows, the octet rule of eight electrons in the valence shell applies.
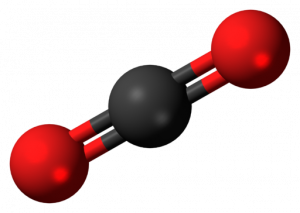
The rows in the periodic table present the elements in order of atomic number and the columns organize elements with similar characteristics, such as the same number of electrons in their valence shells. Columns are often labeled from left to right with Roman numerals I to VIII, and Arabic numerals 1 through 18. The elements in columns I and II have 1 and 2 electrons in their respective valence shells and the elements in columns VI and VII have 6 and 7 electrons in their respective valence shells.
In row 3 and column I, sodium (Na) has 11 protons in the nucleus and 11 electrons in three shells—2 electrons in the inner shell, 8 electrons in the second shell, and 1 electron in the valence shell. To maintain a full outer shell of 8 electrons per the octet rule, sodium readily gives up that 1 electron so there are 10 total electrons. With 11 positively charged protons in the nucleus and 10 negatively charged electrons in two shells, sodium when forming chemical bonds is an ion with an overall net charge of +1.
All elements in column I have a single electron in their valence shell and a valence of 1. These other column I elements also readily give up this single valence electron and thus become ions with a +1 charge. Elements in column II readily give up 2 electrons and end up as ions with a charge of +2. Note that elements in columns I and II which readily give up their valence electrons, often form bonds with elements in columns VI and VII which readily take up these electrons. Elements in columns 3 through 15 are usually involved in covalent bonding. The last column 18 (VIII) contains the noble gases. These elements are chemically inert because the valence shell is already full with 8 electrons, so they do not gain or lose electrons. An example is the noble gas helium which has 2 valence electrons in the first shell. Its valence shell is therefore full. All elements in column VIII possess full valence shells and do not form bonds with other elements.
As seen above, an atom with a net positive or negative charge as a result of gaining or losing electrons is called an ion. In general the elements on the left side of the table lose electrons and become positive ions, called cations because they are attracted to the cathode in an electrical device. The elements on the right side tend to gain electrons. These are called anions because they are attracted to the anode in an electrical device. The elements in the center of the periodic table, columns 3 through 15, do not consistently follow the octet rule. These are called transition elements. A common example is iron, which has a +2 or +3 charge depending on the oxidation state of the element. Oxidized Fe+3 carries a +3 charge and reduced Fe+2 is +2. These two different oxidation states of iron often impart dramatic colors to rocks containing their minerals—the oxidized form producing red colors and the reduced form producing green.
3.1.3.2 Ionic Bonding
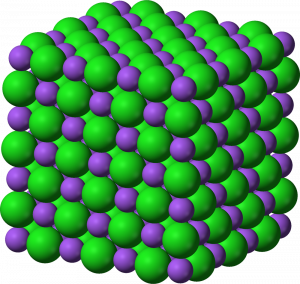
Ionic bonds, also called electron-transfer bonds, are formed by the electrostatic attraction between atoms having opposite charges. Atoms of two opposite charges attract each other electrostatically and form an ionic bond in which the positive ion transfers its electron (or electrons) to the negative ion which takes them up. Through this transfer both atoms thus achieve a full valence shell. For example one atom of sodium (Na+1) and one atom of chlorine (Cl-1) form an ionic bond to make the compound sodium chloride (NaCl). This is also known as the mineral halite or common table salt. Another example is calcium (Ca+2) and chlorine (Cl-1) combining to make the compound calcium chloride (CaCl2). The subscript 2 indicates two atoms of chlorine are ionically bonded to one atom of calcium.
3.1.3.3 Covalent Bonding
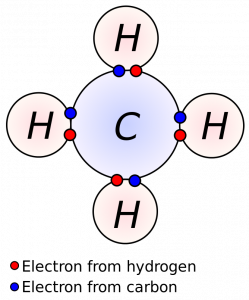
Ionic bonds are usually formed between a metal and a nonmetal. Another type, called a covalent or electron-sharing bond, commonly occurs between nonmetals. Covalent bonds share electrons between ions to complete their valence shells. For example, oxygen (atomic number 8) has 8 electrons—2 in the inner shell and 6 in the valence shell. Gases like oxygen often form diatomic molecules by sharing valence electrons. In the case of oxygen, two atoms attach to each other and share 2 electrons to fill their valence shells to become the common oxygen molecule we breathe (O2). Methane (CH4) is another covalently bonded gas. The carbon atom needs 4 electrons and each hydrogen needs 1. Each hydrogen shares its electron with the carbon to form a molecule as shown in the figure.
Take this quiz to check your comprehension of this section.
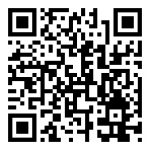
3.2 Formation of Minerals
Minerals form when atoms bond together in a crystalline arrangement. Three main ways this occurs in nature are: 1) precipitation directly from an aqueous (water) solution with a temperature change, 2) crystallization from a magma with a temperature change, and 3) biological precipitation by the action of organisms.
3.2.1 Precipitation from aqueous solution
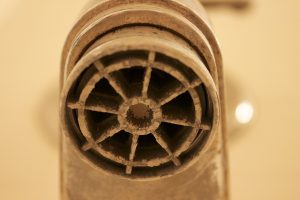
Solutions consist of ions or molecules, known as solutes, dissolved in a medium or solvent. In nature this solvent is usually water. Many minerals can be dissolved in water, such as halite or table salt, which has the composition sodium chloride, NaCl. The Na+1 and Cl-1 ions separate and disperse into the solution.
Precipitation is the reverse process, in which ions in solution come together to form solid minerals. Precipitation is dependent on the concentration of ions in solution and other factors such as temperature and pressure. The point at which a solvent cannot hold any more solute is called saturation. Precipitation can occur when the temperature of the solution falls, when the solute evaporates, or with changing chemical conditions in the solution. An example of precipitation in our homes is when water evaporates and leaves behind a rind of minerals on faucets, shower heads, and drinking glasses.
In nature, changes in environmental conditions may cause the minerals dissolved in water to form bonds and grow into crystals or cement grains of sediment together. In Utah, deposits of tufa formed from mineral-rich springs that emerged into the ice age Lake Bonneville. Now exposed in dry valleys, this porous tufa was a natural insulation used by pioneers to build their homes with a natural protection against summer heat and winter cold. The travertine terraces at Mammoth Hot Springs in Yellowstone Park are another example formed by calcite precipitation at the edges of the shallow spring-fed ponds.
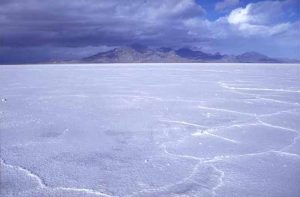
Another example of precipitation occurs in the Great Salt Lake, Utah, where the concentration of sodium chloride and other salts is nearly eight times greater than in the world’s oceans [zotpressInText item="{DU5CMSHJ}" format="%num%" brackets="yes"]. Streams carry salt ions into the lake from the surrounding mountains. With no other outlet, the water in the lake evaporates and the concentration of salt increases until saturation is reached and the minerals precipitate out as sediments. Similar salt deposits include halite and other precipitates, and occur in other lakes like Mono Lake in California and the Dead Sea.
3.2.2 Crystallization from Magma
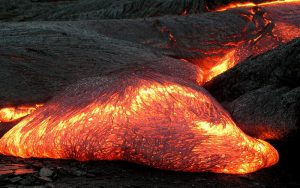
Heat is energy that causes atoms in substances to vibrate. Temperature is a measure of the intensity of the vibration. If the vibrations are violent enough, chemical bonds are broken and the crystals melt releasing the ions into the melt. Magma is molten rock with freely moving ions. When magma is emplaced at depth or extruded onto the surface (then called lava), it starts to cool and mineral crystals can form.
3.2.3 Precipitation by Organisms
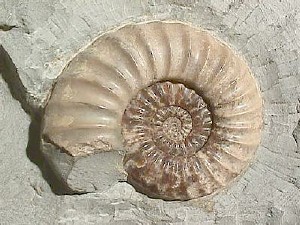
Many organisms build bones, shells, and body coverings by extracting ions from water and precipitating minerals biologically. The most common mineral precipitated by organisms is calcite, or calcium carbonate (CaCO3). Calcite is often precipitated by organisms as a polymorph called aragonite. Polymorphs are crystals with the same chemical formula but different crystal structures. Marine invertebrates such as corals and clams precipitate aragonite or calcite for their shells and structures. Upon death, their hard parts accumulate on the ocean floor as sediments, and eventually may become the sedimentary rock limestone. Though limestone can form inorganically, the vast majority is formed by this biological process. Another example is marine organisms called radiolaria, which are zooplankton that precipitate silica for their microscopic external shells. When the organisms die, the shells accumulate on the ocean floor and can form the sedimentary rock chert. An example of biologic precipitation from the vertebrate world is bone, which is composed mostly of a type of apatite, a mineral in the phosphate group. The apatite found in bones contains calcium and water in its structure and is called hydroxycarbonate apatite, Ca5(PO4)3(OH). As mentioned above, such substances are not technically minerals until the organism dies and these hard parts become fossils.
Take this quiz to check your comprehension of this section.
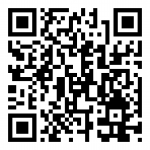
3.3 Silicate Minerals
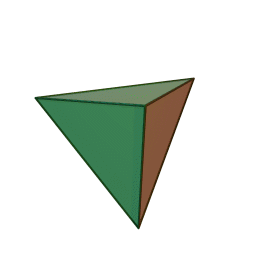
Minerals are categorized based on their composition and structure. Silicate minerals are built around a molecular ion called the silicon-oxygen tetrahedron. A tetrahedron has a pyramid-like shape with four sides and four corners. Silicate minerals form the largest group of minerals on Earth, comprising the vast majority of the Earth’s mantle and crust. Of the nearly four thousand known minerals on Earth, most are rare. There are only a few that make up most of the rocks likely to be encountered by surface dwelling creatures like us. These are generally called the rock-forming minerals.
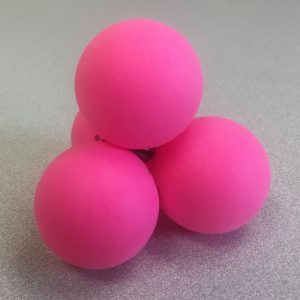
The silicon-oxygen tetrahedron (SiO4) consists of a single silicon atom at the center and four oxygen atoms located at the four corners of the tetrahedron. Each oxygen ion has a -2 charge and the silicon ion has a +4 charge. The silicon ion shares one of its four valence electrons with each of the four oxygen ions in a covalent bond to create a symmetrical geometric four-sided pyramid figure. Only half of the oxygen’s valence electrons are shared, giving the silicon-oxygen tetrahedron an ionic charge of -4. This silicon-oxygen tetrahedron forms bonds with many other combinations of ions to form the large group of silicate minerals.
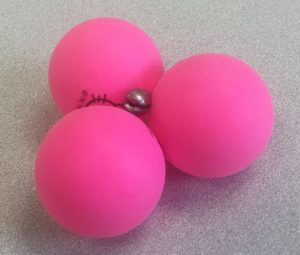
The silicon ion is much smaller than the oxygen ions (see the figures) and fits into a small space in the center of the four large oxygen ions, seen if the top ball is removed (as shown in the figure to the right). Because only one of the valence electrons of the corner oxygens is shared, the silicon-oxygen tetrahedron has chemically active corners available to form bonds with other silica tetrahedra or other positively charged ions such as Al+3, Fe+2,+3, Mg+2, K+1, Na+1, and Ca+2. Depending on many factors, such as the original magma chemistry, silica-oxygen tetrahedra can combine with other tetrahedra in several different configurations. For example, tetrahedra can be isolated, attached in chains, sheets, or three dimensional structures. These combinations and others create the chemical structure in which positively charged ions can be inserted for unique chemical compositions forming silicate mineral groups.
3.3.1 The dark ferromagnesian silicates
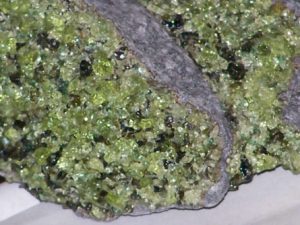
The Olivine Family
Olivine is the primary mineral component in mantle rock such as peridotite and basalt. It is characteristically green when not weathered. The chemical formula is (Fe,Mg)2SiO4. As previously described, the comma between iron (Fe) and magnesium (Mg) indicates these two elements occur in a solid solution. Not to be confused with a liquid solution, a solid solution occurs when two or more elements have similar properties and can freely substitute for each other in the same location in the crystal structure.
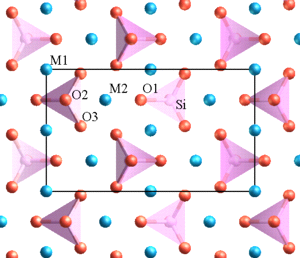
Olivine is referred to as a mineral family because of the ability of iron and magnesium to substitute for each other. Iron and magnesium in the olivine family indicates a solid solution forming a compositional series within the mineral group which can form crystals of all iron as one end member and all mixtures of iron and magnesium in between to all magnesium at the other end member. Different mineral names are applied to compositions between these end members. In the olivine series of minerals, the iron and magnesium ions in the solid solution are about the same size and charge, so either atom can fit into the same location in the growing crystals. Within the cooling magma, the mineral crystals continue to grow until they solidify into igneous rock. The relative amounts of iron and magnesium in the parent magma determine which minerals in the series form. Other rarer elements with similar properties to iron or magnesium, like manganese (Mn), can substitute into the olivine crystalline structure in small amounts. Such ionic substitutions in mineral crystals give rise to the great variety of minerals and are often responsible for differences in color and other properties within a group or family of minerals. Olivine has a pure iron end-member (called fayalite) and a pure magnesium end-member (called forsterite). Chemically, olivine is mostly silica, iron, and magnesium and therefore is grouped among the dark-colored ferromagnesian (iron=ferro, magnesium=magnesian) or mafic minerals, a contraction of their chemical symbols Ma and Fe. Mafic minerals are also referred to as dark-colored ferromagnesian minerals. Ferro means iron and magnesian refers to magnesium. Ferromagnesian silicates tend to be more dense than non-ferromagnesian silicates. This difference in density ends up being important in controlling the behavior of the igneous rocks that are built from these minerals: whether a tectonic plate subducts or not is largely governed by the density of its rocks, which are in turn controlled by the density of the minerals that comprise them.
The crystal structure of olivine is built from independent silica tetrahedra. Minerals with independent tetrahedral structures are called neosilicates (or orthosilicates). In addition to olivine, other common neosilicate minerals include garnet, topaz, kyanite, and zircon.
Two other similar arrangements of tetrahedra are close in structure to the neosilicates and grade toward the next group of minerals, the pyroxenes. In a variation on independent tetrahedra called sorosilicates, there are minerals that share one oxygen between two tetrahedra, and include minerals like pistachio-green epidote, a gemstone. Another variation are the cyclosilicates, which as the name suggests, consist of tetrahedral rings, and include gemstones such as beryl, emerald, aquamarine, and tourmaline
3.3.2 Pyroxene Family
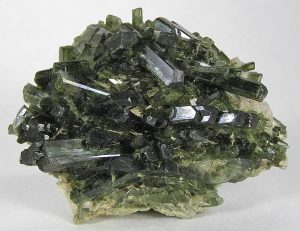

Pyroxene is another family of dark ferromagnesian minerals, typically black or dark green in color. Members of the pyroxene family have a complex chemical composition that includes iron, magnesium, aluminum, and other elements bonded to polymerized silica tetrahedra. Polymers are chains, sheets, or three-dimensional structures, and are formed by multiple tetrahedra covalently bonded via their corner oxygen atoms. Pyroxenes are commonly found in mafic igneous rocks such as peridotite, basalt, and gabbro, as well as metamorphic rocks like eclogite and blue schist.
Pyroxenes are built from long, single chains of polymerized silica tetrahedra in which tetrahedra share two corner oxygens. The silica chains are bonded together into the crystal structures by metal cations. A common member of the pyroxene family is augite, itself containing several solid solution series with a complex chemical formula (Ca,Na)(Mg,Fe,Al,Ti)(Si,Al)2O6 that gives rise to a number of individual mineral names.
This single-chain crystalline structure bonds with many elements, which can also freely substitute for each other. The generalized chemical composition for pyroxene is XZ(Al,Si)2O6. X represents the ions Na, Ca, Mg, or Fe, and Z represents Mg, Fe, or Al. These ions have similar ionic sizes, which allows many possible substitutions among them. Although the cations may freely substitute for each other in the crystal, they carry different ionic charges that must be balanced out in the final crystalline structure. For example Na has a charge of +1, but Ca has charge of +2. If a Na+ ion substitutes for a Ca+2 ion, it creates an unequal charge that must be balanced by other ionic substitutions elsewhere in the crystal. Note that ionic size is more important than ionic charge for substitutions to occur in solid solution series in crystals.
3.3.3 Amphibole Family
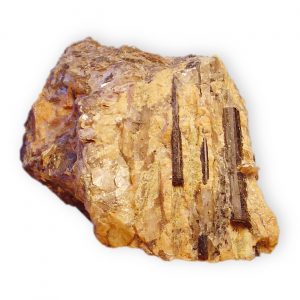
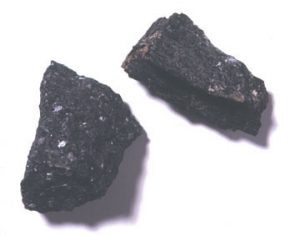
Amphibole minerals are built from polymerized double silica chains and they are also referred to as inosilicates. Imagine two pyroxene chains that connect together by sharing a third oxygen on each tetrahedra. Amphiboles are usually found in igneous and metamorphic rocks and typically have a long-bladed crystal habit. The most common amphibole, hornblende, is usually black; however, they come in a variety of colors depending on their chemical composition. The metamorphic rock, amphibolite, is primarily composed of amphibole minerals.
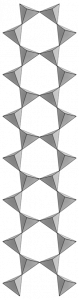
Amphiboles are composed of iron, magnesium, aluminum, and other cations bonded with silica tetrahedra. These dark ferromagnesian minerals are commonly found in gabbro, baslt, diorite, and often form the black specks in granite. Their chemical formula is very complex and generally written as (RSi4O11)2, where R represents many different cations. For example, it can also be written more exactly as AX2Z5((Si,Al,Ti)8O22)(OH,F,Cl,O)2. In this formula A may be Ca, Na, K, Pb, or blank; X equals Li, Na, Mg, Fe, Mn, or Ca; and Z is Li, Na, Mg, Fe, Mn, Zn, Co, Ni, Al, Cr, Mn, V, Ti, or Zr. The substitutions create a wide variety of colors such as green, black, colorless, white, yellow, blue, or brown. Amphibole crystals can also include hydroxide ions (OH-), which occurs from an interaction between the growing minerals and water dissolved in magma.
3.3.4 Sheet Silicates
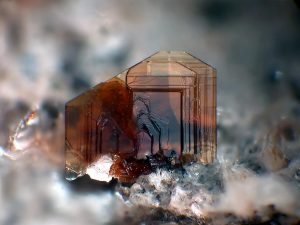
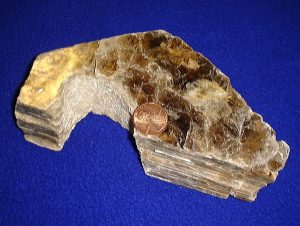
Sheet silicates are built from tetrahedra which share all three of their bottom corner oxygens thus forming sheets of tetrahedra with their top corners available for bonding with other atoms. Micas and clays are common types of sheet silicates, also known as phyllosilicates. Mica minerals are usually found in igneous and metamorphic rocks, while clay minerals are more often found in sedimentary rocks. Two frequently found micas are dark-colored biotite, frequently found in granite, and light-colored muscovite, found in the metamorphic rock called schist.
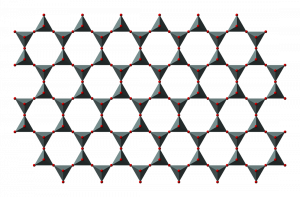
Chemically, sheet silicates usually contain silicon and oxygen in a 2:5 ratio (Si4O10). Micas contain mostly silica, aluminum, and potassium. Biotite mica has more iron and magnesium and is considered a ferromagnesian silicate mineral. Muscovite micas belong to the felsic silicate minerals. Felsic is a contraction formed from feldspar, the dominant mineral in felsic rocks.
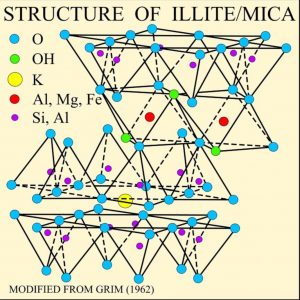
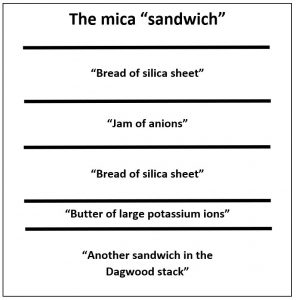
The illustration of the crystalline structure of mica shows the corner O atoms bonded with K, Al, Mg, Fe, and Si atoms, forming polymerized sheets of linked tetrahedra, with an octahedral layer of Fe, Mg, or Al, between them. The yellow potassium ions form Van der Waals bonds (attraction and repulsion between atoms, molecules, and surfaces) and hold the sheets together. Van der Waals bonds differ from covalent and ionic bonds, and exist here between the sandwiches, holding them together into a stack of sandwiches. The Van der Waals bonds are weak compared to the bonds within the sheets, allowing the sandwiches to be separated along the potassium layers. This gives mica its characteristic property of easily cleaving into sheets.
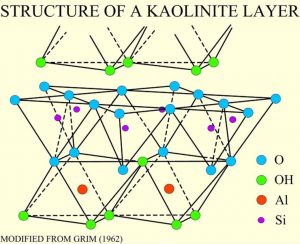
Clays minerals occur in sediments formed by the weathering of rocks and are another family of silicate minerals with a tetrahedral sheet structure. Clay minerals form a complex family, and are an important component of many sedimentary rocks. Other sheet silicates include serpentine and chlorite, found in metamorphic rocks.
Clay minerals are composed of hydrous aluminum silicates. One type of clay, kaolinite, has a structure like an open-faced sandwich, with the bread being a single layer of silicon-oxygen tetrahedra and a layer of aluminum as the spread in an octahedral configuration with the top oxygens of the sheets.
3.3.5 Framework Silicates
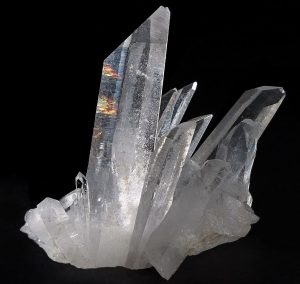
Quartz and feldspar are the two most abundant minerals in the continental crust. In fact, feldspar itself is the single most abundant mineral in the Earth’s crust. There are two types of feldspar, one containing potassium and abundant in felsic rocks of the continental crust, and the other with sodium and calcium abundant in the mafic rocks of oceanic crust. Together with quartz, these minerals are classified as framework silicates. They are built with a three-dimensional framework of silica tetrahedra in which all four corner oxygens are shared with adjacent tetrahedra. Within these frameworks in feldspar are holes and spaces into which other ions like aluminum, potassium, sodium, and calcium can fit giving rise to a variety of mineral compositions and mineral names.
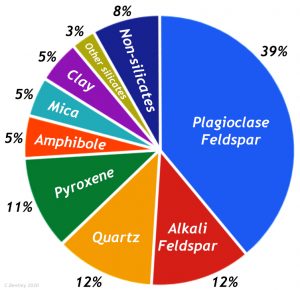
Feldspars are usually found in igneous rocks, such as granite, rhyolite, and basalt as well as metamorphic rocks and detrital sedimentary rocks. Detrital sedimentary rocks are composed of mechanically weathered rock particles, like sand and gravel. Quartz is especially abundant in detrital sedimentary rocks because it is very resistant to disintegration by weathering. While quartz is the most abundant mineral on the Earth's surface, due to its durability, the feldspar minerals are the most abundant minerals in the Earth's crust, comprising roughly 50% of the total minerals that make up the crust.
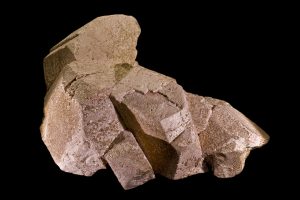
Quartz is composed of pure silica, SiO2, with the tetrahedra arranged in a three dimensional framework. Impurities consisting of atoms within this framework give rise to many varieties of quartz among which are gemstones like amethyst, rose quartz, and citrine. Feldspars are mostly silica with aluminum, potassium, sodium, and calcium. Orthoclase feldspar (KAlSi3O8), also called potassium feldspar or K-spar, is made of silica, aluminum, and potassium. Quartz and orthoclase feldspar are felsic minerals. Felsic is the compositional term applied to continental igneous minerals and rocks that contain an abundance of silica. Another feldspar is plagioclase with the formula (Ca,Na)AlSi3O8, the solid solution (Ca,Na) indicating a series of minerals, one end of the series with calcium CaAl2Si2O8, called anorthite, and the other end with sodium NaAlSi3O8, called albite. Note how the mineral accommodates the substitution of Ca++ and Na+. Minerals in this solid solution series have different mineral names.
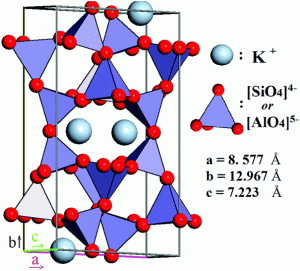
Note that aluminum, which has a similar ionic size to silicon, can substitute for silicon inside the tetrahedra (see figure). Because potassium ions are so much larger than sodium and calcium ions, which are very similar in size, the inability of the crystal lattice to accommodate both potassium and sodium/calcium gives rise to the two families of feldspar, orthoclase and plagioclase respectively. Framework silicates are called tectosilicates and include the alkali metal-rich feldspathoids and zeolites.
Take this quiz to check your comprehension of this section.
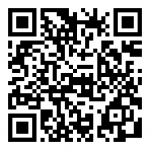
3.4 Non-Silicate Minerals
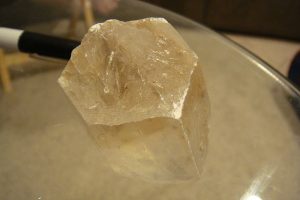
The crystal structure of non-silicate minerals (see table) does not contain silica-oxygen tetrahedra. Many non-silicate minerals are economically important and provide metallic resources such as copper, lead, and iron. They also include valuable non-metallic products such as salt, construction materials, and fertilizer.
Mineral Group | Examples | Formula | Uses |
Native elements | gold, silver, copper | Au, Ag, Cu | Jewelry, coins, industry |
Carbonates | calcite, dolomite | CaCO3, CaMg(CO3)2 | Lime, Portland cement |
Oxides | hematite, magnetite, bauxite | Fe2O3, Fe3O4, a mixture of aluminum oxides | Ores of iron & aluminum, pigments |
Halides | halite, sylvite | NaCl, KCl | Table salt, fertilizer |
Sulfides | galena, chalcopyrite, cinnabar | PbS, CuFeS2, HgS | Ores of lead, copper, mercury |
Sulphates | gypsum, epsom salts | CaSo4·2H2O, MgSO4·7H2O | Sheetrock, therapeutic soak |
Phosphates | apatite | Ca5(PO4)3(F,Cl,OH) | Fertilizer, teeth, bones |
Common non-silicate mineral groups.
3.4.1 Carbonates
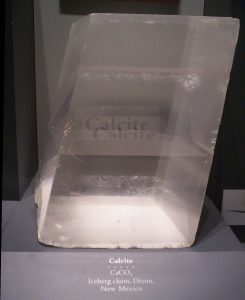
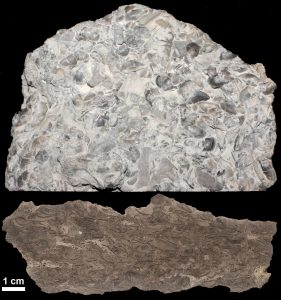
Calcite (CaCO3) and dolomite (CaMg(CO3)2) are the two most frequently occurring carbonate minerals, and usually occur in sedimentary rocks, such as limestone and dolostone rocks, respectively. Some carbonate rocks, such calcite and dolomite, are formed via evaporation and precipitation. However, most carbonate-rich rocks, such as limestone, are created by the lithification of fossilized marine organisms. These organisms, including those we can see and many microscopic organisms, have shells or exoskeletons consisting of calcium carbonate (CaCO3). When these organisms die, their remains accumulate on the floor of the water body in which they live and the soft body parts decompose and dissolve away. The calcium carbonate hard parts become included in the sediments, eventually becoming the sedimentary rock called limestone. While limestone may contain large, easy to see fossils, most limestones contain the remains of microscopic creatures and thus originate from biological processes.
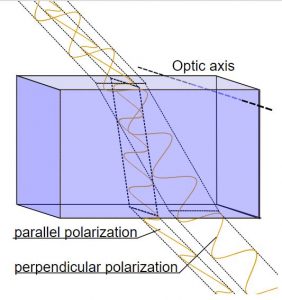
Calcite crystals show an interesting property called birefringence, meaning they polarize light into two wave components vibrating at right angles to each other. As the two light waves pass through the crystal, they travel at different velocities and are separated by refraction into two different travel paths. In other words, the crystal produces a double image of objects viewed through it. Because they polarize light, calcite crystals are used in special petrographic microscopes for studying minerals and rocks.
Many non-silicate minerals are referred to as salts. The term salts used here refers to compounds made by replacing the hydrogen in natural acids. The most abundant natural acid is carbonic acid that forms by the solution of carbon dioxide in water. Carbonate minerals are salts built around the carbonate ion (CO3-2) where calcium and/or magnesium replace the hydrogen in carbonic acid (H2CO3). Calcite and a closely related polymorph aragonite are secreted by organisms to form shells and physical structures like corals. Many such creatures draw both calcium and carbonate from dissolved bicarbonate ions (HCO3-) in ocean water. As seen in the mineral identification section below, calcite is easily dissolved in acid and thus effervesces in dilute hydrochloric acid (HCl). Small dropper bottles of dilute hydrochloric acid are often carried by geologists in the field as well as used in mineral identification labs.
Other salts include halite (NaCl) in which sodium replaces the hydrogen in hydrochloric acid and gypsum (Ca[SO4] • 2 H2O) in which calcium replaces the hydrogen in sulfuric acid. Note that some water molecules are also included in the gypsum crystal. Salts are often formed by evaporation and are called evaporite minerals.
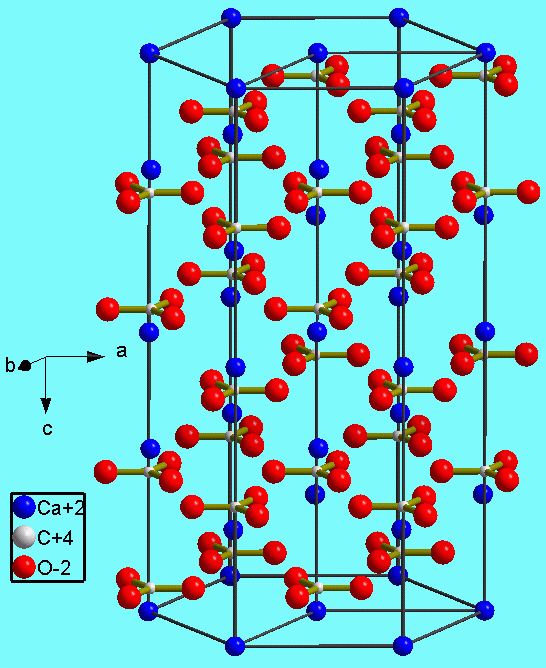
The figure shows the crystal structure of calcite (CaCO3). Like silicon, carbon has four valence electrons. The carbonate unit consists of carbon atoms (tiny white dots) covalently bonded to three oxygen atoms (red), one oxygen sharing two valence electrons with the carbon and the other two sharing one valence electron each with the carbon, thus creating triangular units with a charge of -2. The negatively charged carbonate unit forms an ionic bond with the Ca ion (blue), which as a charge of +2.
3.4.2 Oxides, Halides, and Sulfides
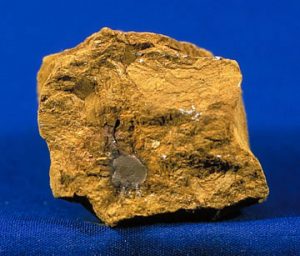
After carbonates, the next most common non-silicate minerals are the oxides, halides, and sulfides.
Oxides consist of metal ions covalently bonded with oxygen. The most familiar oxide is rust, which is a combination of iron oxides (Fe2O3) and hydrated oxides. Hydrated oxides form when iron is exposed to oxygen and water. Iron oxides are important for producing metallic iron. When iron oxide or ore is smelted, it produces carbon dioxide (CO2) and metallic iron.
The red color in rocks is usually due to the presence of iron oxides. For example, the red sandstone cliffs in Zion National Park and throughout Southern Utah consist of white or colorless grains of quartz coated with iron oxide which serve as cementing agents holding the grains together.
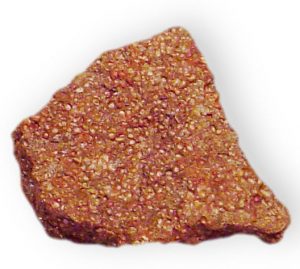
Other iron oxides include limonite, magnetite, and hematite. Hematite occurs in many different crystal forms. The massive form shows no external structure. Botryoidal hematite shows large concentric blobs. Specular hematite looks like a mass of shiny metallic crystals. Oolitic hematite looks like a mass of dull red fish eggs. These different forms of hematite are polymorphs and all have the same formula, Fe2O3.
Other common oxide minerals include:
- ice (H2O), an oxide of hydrogen
- bauxite (Al2H2O4), hydrated oxides of aluminum, an ore for producing metallic aluminum
- corundum (Al2O3), which includes ruby and sapphire gemstones.
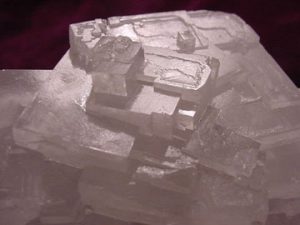
The halides consist of halogens in column VII, usually fluorine or chlorine, ionically bonded with sodium or other cations. These include halite or sodium chloride (NaCl), common table salt; sylvite or potassium chloride (KCl); and fluorite or calcium fluoride (CaF2).
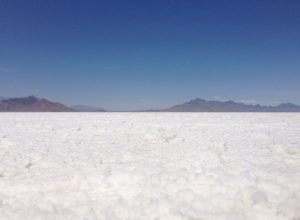
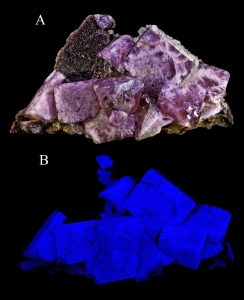
Halide minerals usually form from the evaporation of sea water or other isolated bodies of water. A well-known example of halide mineral deposits created by evaporation is the Bonneville Salt Flats, located west of the Great Salt Lake in Utah (see figure).
Many important metal ores are sulfides, in which metals are bonded to sulfur. Significant examples include: galena (lead sulfide), sphalerite (zinc sulfide), pyrite
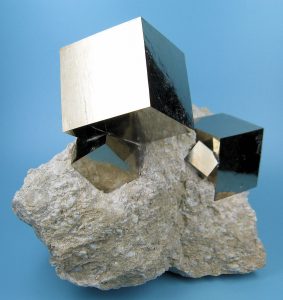
(iron sulfide, sometimes called “fool's gold”), and chalcopyrite (iron-copper sulfide). Sulfides are well known for being important ore minerals. For example, galena is the main source of lead, sphalerite is the main source of zinc, and chalcopyrite is the main copper ore mineral mined in porphyry deposits like the Bingham mine (see chapter 16). The largest sources of nickel, antimony, molybdenum, arsenic, and mercury are also sulfides.
3.4.3 Sulfates
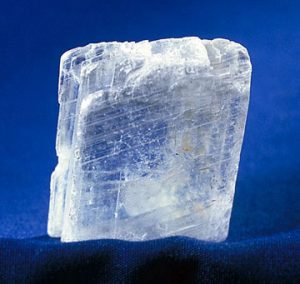
Sulfate minerals contain a metal ion, such as calcium, bonded to a sulfate ion. The sulfate ion is a combination of sulfur and oxygen (SO4-2). The sulfate mineral gypsum (CaSO4ᐧ2H2O) is used in construction materials such as plaster and drywall. Gypsum is often formed from evaporating water and usually contains water molecules in its crystalline structure. The ᐧ2H2O in the formula indicates the water molecules are whole H2O. This is different from minerals like amphibole, which contain a hydroxide ion (OH-) that is derived from water, but is missing a hydrogen ion (H+). The calcium sulfate without water is a different mineral than gypsum called anhydrite (CaSO4).
3.4.4 Phosphates
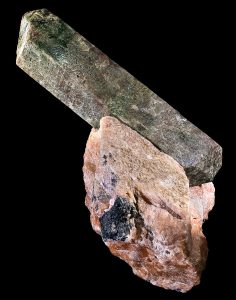
Phosphate minerals have a tetrahedral phosphate unit (PO4-3) combined with various anions and cations. In some cases arsenic or vanadium can substitute for phosphorus. Phosphates are an important ingredient of fertilizers as well as detergents, paint, and other products. The best known phosphate mineral is apatite, Ca5(PO4)3(F,Cl,OH), variations of which are found in teeth and bones. The gemstone turquoise [CuAl6(PO4)4(OH)8·4H2O ] is a copper-rich phosphate mineral that, like gypsum, contains water molecules.
3.4.5 Native Element Minerals
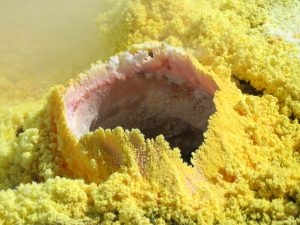
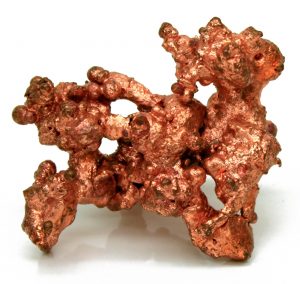
Native element minerals, usually metals, occur in nature in a pure or nearly pure state. Gold is an example of a native element mineral; it is not very reactive and rarely bonds with other elements so it is usually found in an isolated or pure state. The non-metallic and poorly-reactive mineral carbon is often found as a native element, such as graphite and diamonds. Mildly reactive metals like silver, copper, platinum, mercury, and sulfur sometimes occur as native element minerals. Reactive metals such as iron, lead, and aluminum almost always bond to other elements and are rarely found in a native state.
Take this quiz to check your comprehension of this section.
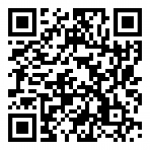
3.5 Identifying Minerals
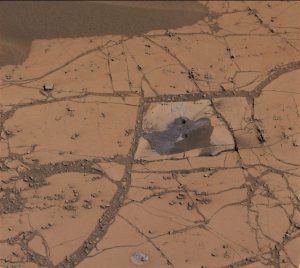
Geologists identify minerals by their physical properties. In the field, where geologists may have limited access to advanced technology and powerful machines, they can still identify minerals by testing several physical properties: luster and color, streak, hardness, crystal habit, cleavage and fracture, and some special properties. Only a few common minerals make up the majority of Earth's rocks and are usually seen as small grains in rocks. Of the several properties used for identifying minerals, it is good to consider which will be most useful for identifying them in small grains surrounded by other minerals.
3.5.1 Luster and Color
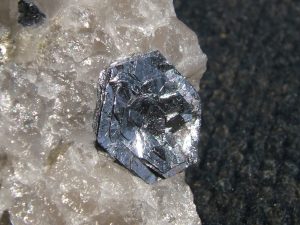
The first thing to notice about a mineral is its surface appearance, specifically luster and color. Luster describes how the mineral looks. Metallic luster looks like a shiny metal such as chrome, steel, silver, or gold. Submetallic luster has a duller appearance. Pewter, for example, shows submetallic luster.
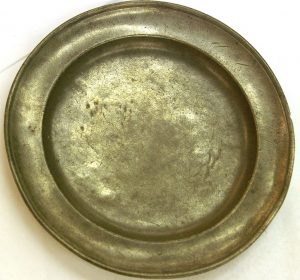
Nonmetallic luster doesn’t look like a metal and may be described as vitreous (glassy), earthy, silky, pearly, and other surface qualities. Nonmetallic minerals may be shiny, although their vitreous shine is different from metallic luster. See the table for descriptions and examples of nonmetallic luster.
Luster | Image | Description |
---|---|---|
Vitreous/glassy |
![]() |
Surface is shiny like glass |
Earthy/dull |
![]() |
Dull, like dried mud or clay |
Silky |
![]() |
Soft shine like silk fabric |
Pearly |
![]() |
Like the inside of a clam shell or mother-of-pearl |
Submetallic |
![]() |
Has the appearance of dull metal, like pewter. These minerals would usually still be considered metallic. Submetallic appearance can occur in metallic minerals because of weathering. |
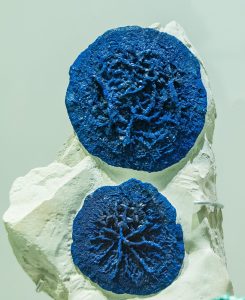
Surface color may be helpful in identifying minerals, although it can be quite variable within the same mineral family. Mineral colors are affected by the main elements as well as impurities in the crystals. These impurities may be rare elements—like manganese, titanium, chromium, or lithium—even other molecules that are not normally part of the mineral formula. For example, the incorporation of water molecules gives quartz, which is normally clear, a milky color.
Some minerals predominantly show a single color. Malachite and azurite are green and blue, respectively, because of their copper content. Other minerals have a predictable range of colors due to elemental substitutions, usually via a solid solution. Feldspars, the most abundant minerals in the earth’s crust, are complex, have solid solution series, and present several colors including pink, white, green, gray and others. Other minerals also come in several colors, influenced by trace amounts of several elements. The same element may show up as different colors, in different minerals. With notable exceptions, color is usually not a definitive property of minerals. For identifying many minerals. a more reliable indicator is streak, which is the color of the powdered mineral.
3.5.2 Streak
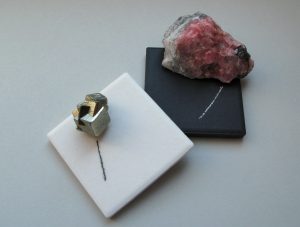
Streak examines the color of a powdered mineral, and can be seen when a mineral sample is scratched or scraped on an unglazed porcelain streak plate. A paper page in a field notebook may also be used for the streak of some minerals. Minerals that are harder than the streak plate will not show streak, but will scratch the porcelain. For these minerals, a streak test can be obtained by powdering the mineral with a hammer and smearing the powder across a streak plate or notebook paper.
While mineral surface colors and appearances may vary, their streak colors can be diagnostically useful. An example of this property is seen in the iron-oxide mineral hematite. Hematite occurs in a variety of forms, colors and lusters, from shiny metallic silver to earthy red-brown, and different physical appearances. A hematite streak is consistently reddish brown, no matter what the original specimen looks like. Iron sulfide or pyrite, is a brassy metallic yellow. Commonly named fool’s gold, pyrite has a characteristic black to greenish-black streak.
3.5.3 Hardness
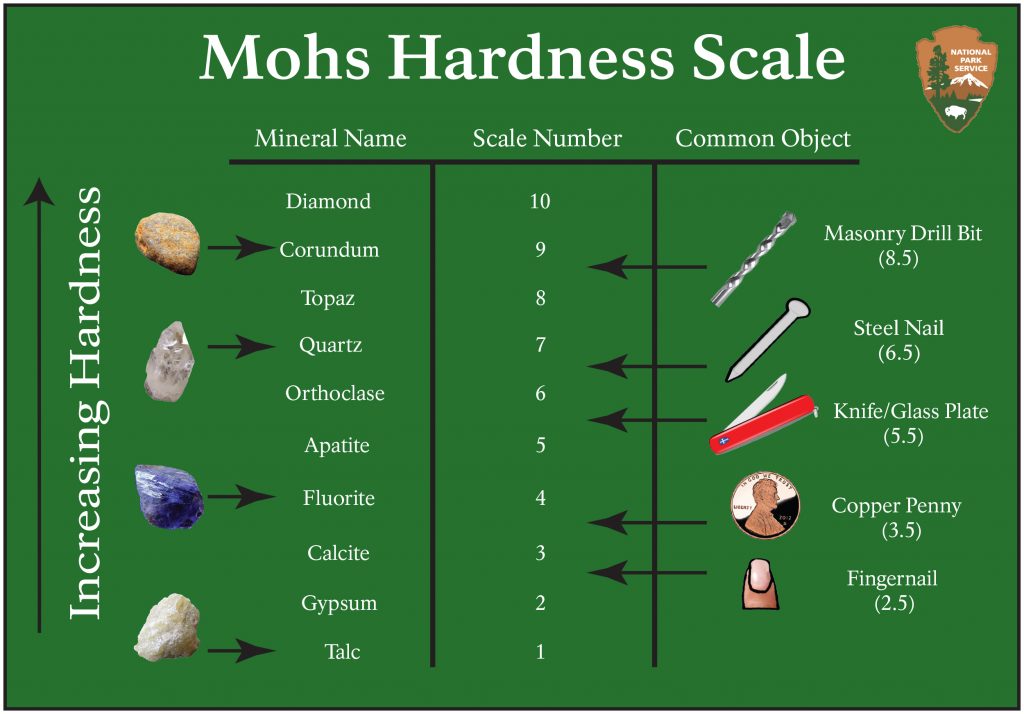
Hardness measures the ability of a mineral to scratch other substances. The Mohs Hardness Scale gives a number showing the relative scratch-resistance of minerals when compared to a standardized set of minerals of increasing hardness. The Mohs scale was developed by German geologist Fredrick Mohs in the early 20th century, although the idea of identifying minerals by hardness goes back thousands of years. Mohs hardness values are determined by the strength of a mineral’s atomic bonds.
The figure shows the minerals associated with specific hardness values, together with some common items readily available for use in field testing and mineral identification. The hardness values run from 1 to 10, with 10 being the hardest; however, the scale is not linear. Diamond defines a hardness of 10 and is actually about four times harder than corundum, which is 9. A steel pocketknife blade, which has a hardness value of 5.5, separates between hard and soft minerals on many mineral identification keys.
3.5.4 Crystal Habit
Minerals can be identified by crystal habit, how their crystals grow and appear in rocks. Crystal shapes are determined by the arrangement of the atoms within the crystal structure. For example, a cubic arrangement of atoms gives rise to a cubic-shaped mineral crystal. Crystal habit refers to typically observed shapes and characteristics; however, they can be affected by other minerals crystallizing in the same rock. When minerals are constrained so they do not develop their typical crystal habit, they are called anhedral. Subhedral crystals are partially formed shapes. For some minerals characteristic crystal habit is to grow crystal faces even when surrounded by other crystals in rock. An example is garnet. Minerals grown freely where the crystals are unconstrained and can take characteristic shapes often form crystal faces. A euhedral crystal has a perfectly formed, unconstrained shape. Some minerals crystallize in such tiny crystals, they do not show a specific crystal habit to the naked eye. Other minerals, like pyrite, can have an array of different crystal habits, including cubic, dodecahedral, octahedral, and massive. The table lists typical crystal habits of various minerals.
Habit | Image | Examples |
---|---|---|
Bladed
long and flat crystals |
![]() |
kyanite, amphibole, gypsum |
Botryoidal/mammillary
blobby, circular crystals |
![]() |
hematite, malachite, smithsonite |
Coating/laminae/druse
crystals that are small and coat surfaces |
![]() |
quartz, calcite, malachite, azurite |
Cubic
cube-shaped crystals |
![]() |
pyrite, galena, halite |
Dodecahedral
12-sided polygon shapes |
![]() |
garnet, pyrite |
Dendritic
branching crystals |
![]() |
Mn-oxides, copper, gold |
Equant
crystals that do not have a long direction |
![]() |
olivine, garnet, pyroxene |
Fibrous
thin, very long crystals |
![]() |
serpentine, amphibole, zeolite |
Layered, sheets
stacked, very thin, flat crystals |
![]() |
mica (biotite, muscovite, etc.) |
Lenticular/platy
crystals that are plate-like |
![]() |
selenite roses, wulfenite, calcite |
Hexagonal
crystals with six sides |
![]() |
quartz, hanksite, corundum |
Massive/granular
Crystals with no obvious shape, microscopic crystals |
![]() |
limonite, pyrite, azurite, bornite |
Octahedral
4-sided double pyramid crystals |
![]() |
diamond, fluorite, magnetite, pyrite |
Prismatic/columnar
very long, cylindrical crystals |
![]() |
tourmaline, beryl, barite |
Radiating
crystals that grow from a point and fan out |
![]() |
pyrite "suns", pyrophyllite |
Rhombohedral
crystals shaped like slanted cubes |
![]() |
calcite, dolomite |
Tabular/blocky/stubby
sharp-sided crystals with no long direction |
![]() |
feldspar, pyroxene, calcite |
Tetrahedral
three-sided, pyramid-shaped crystals |
![]() |
magnetite, spinel, tetrahedrite |
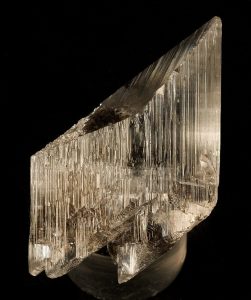
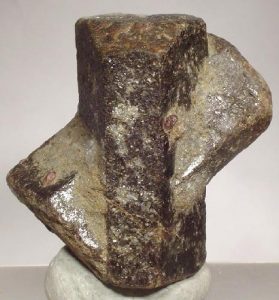
Another crystal habit that may be used to identify minerals is striations, which are dark and light parallel lines on a crystal face. Twinning is another, which occurs when the crystal structure replicates in mirror images along certain directions in the crystal.
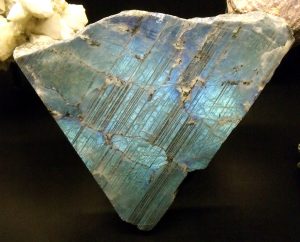
Striations and twinning are related properties in some minerals including plagioclase feldspar. Striations are optical lines on a cleavage surface. Because of twinning in the crystal, striations show up on one of the two cleavage faces of the plagioclase crystal.
3.5.5 Cleavage and Fracture
Minerals often show characteristic patterns of breaking along specific cleavage planes or show characteristic fracture patterns. Cleavage planes are smooth, flat, parallel planes within the crystal. The cleavage planes may show as reflective surfaces on the crystal, as parallel cracks that penetrate into the crystal, or show on the edge or side of the crystal as a series of steps like rice terraces. Cleavage arises in crystals where the atomic bonds between atomic layers are weaker along some directions than others, meaning they will break preferentially along these planes. Because they develop on atomic surfaces in the crystal, cleavage planes are optically smooth and reflect light, although the actual break on the crystal may appear jagged or uneven. In such cleavages, the cleavage surface may appear like rice terraces on a mountainside that all reflect sunlight from a particular sun angle. Some minerals have a strong cleavage, some minerals only have weak cleavage or do not typically demonstrate cleavage.
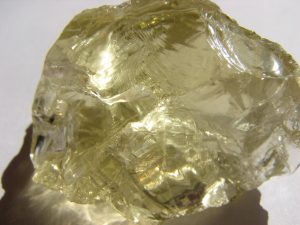
For example, quartz and olivine rarely show cleavage and typically break into conchoidal fracture patterns.
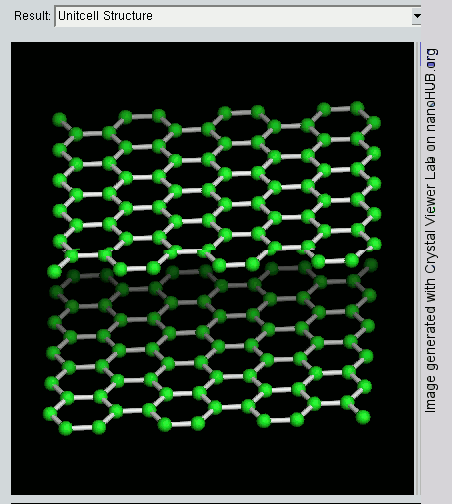
Graphite has its carbon atoms arranged into layers with relatively strong bonds within the layer and very weak bonds between the layers. Thus graphite cleaves readily between the layers and the layers slide easily over one another giving graphite its lubricating quality.
Mineral fracture surfaces may be rough and uneven or they may be show conchoidal fracture. Uneven fracture patterns are described as irregular, splintery, fibrous. A conchoidal fracture has a smooth, curved surface like a shallow bowl or conch shell, often with curved ridges. Natural volcanic glass, called obsidian, breaks with this characteristic conchoidal pattern
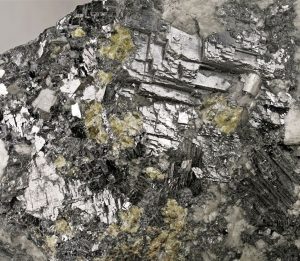
To work with cleavage, it is important to remember that cleavage is a result of bonds separating along planes of atoms in the crystal structure. On some minerals, cleavage planes may be confused with crystal faces. This will usually not be an issue for crystals of minerals that grew together within rocks. The act of breaking the rock to expose a fresh face will most likely break the crystals along cleavage planes. Some cleavage planes are parallel with crystal faces but many are not. Cleavage planes are smooth, flat, parallel planes within the crystal. The cleavage planes may show as parallel cracks that penetrate into the crystal (see amphibole below), or show on the edge or side of the crystal as a series of steps like rice terraces. For some minerals characteristic crystal habit is to grow crystal faces even when surrounded by other crystals in rock. An example is garnet. Minerals grown freely where the crystals are unconstrained and can take characteristic shapes often form crystal faces (see quartz below).
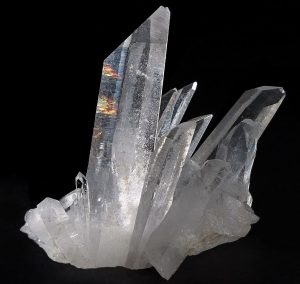
In some minerals, distinguishing cleavage planes from crystal faces may be challenging for the student. Understanding the nature of cleavage and referring to the number of cleavage planes and cleavage angles on identification keys should provide the student with enough information to distinguish cleavages from crystal faces. Cleavage planes may show as multiple parallel cracks or flat surfaces on the crystal. Cleavage planes may be expressed as a series of steps like terraced rice paddies. See the cleavage surfaces on galena above or plagioclase below. Cleavage planes arise from the tendency of mineral crystals to break along specific planes of weakness within the crystal favored by atomic arrangements. The number of cleavage planes, the quality of the cleavage surfaces, and the angles between them are diagnostic for many minerals and cleavage is one of the most useful properties for identifying minerals. Learning to recognize cleavage is an especially important and useful skill in studying minerals.
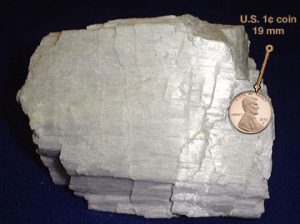
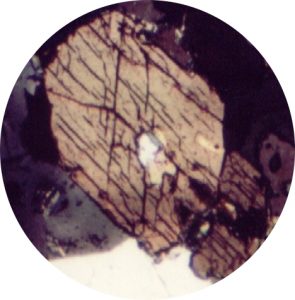
As an identification property of minerals, cleavage is usually given in terms of the quality of the cleavage (perfect, imperfect, or none), the number of cleavage surfaces, and the angles between the surfaces. The most common number of cleavage plane directions in the common rock-forming minerals are: one perfect cleavage (as in mica), two cleavage planes (as in feldspar, pyroxene, and amphibole), and three cleavage planes (as in halite, calcite, and galena). One perfect cleavage (as in mica) develops on the top and bottom of the mineral specimen with many parallel cracks showing on the sides but no angle of intersection. Two cleavage planes intersect at an angle. Common cleavage angles are 60°, 75°, 90°, and 120°. Amphibole has two cleavage planes at 60° and 120°. Galena and halite have three cleavage planes at 90° (cubic cleavage). Calcite cleaves readily in three directions producing a cleavage figure called a rhomb that looks like a cube squashed over toward one corner giving rise to the approximately 75° cleavage angles. Pyroxene has an imperfect cleavage with two planes at 90°.
Cleavages on common rock-forming minerals
- Quartz—none (conchoidal fracture)
- Olivine—none (conchoidal fracture)
- Mica—1 perfect
- Feldspar—2 perfect at 90°
- Pyroxene—2 imperfect at 90°
- Amphibole—2 perfect at 60°/120°
- Calcite—3 perfect at approximately 75°
- Halite, galena, pyrite—3 perfect at 90°
3.5.6 Special Properties
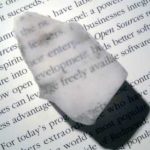
Special properties are unique and identifiable characteristics used to identify minerals or that allow some minerals to be used for special purposes. Ulexite has a fiber-optic property that can project images through the crystal like a high-definition television screen (see figure). A simple identifying special property is taste, such as the salty flavor of halite or common table salt (NaCl). Sylvite is potassium chloride (KCl) and has a more bitter taste.
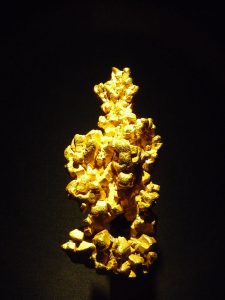
Another property geologists may use to identify minerals is a property related to density called specific gravity. Specific gravity measures the weight of a mineral specimen relative to the weight of an equal volume of water. The value is expressed as a ratio between the mineral and water weights. To measure specific gravity, a mineral specimen is first weighed in grams then submerged in a graduated cylinder filled with pure water at room temperature. The rise in water level is noted using the cylinder’s graduated scale. Since the weight of water at room temperature is 1 gram per cubic centimeter, the ratio of the two weight numbers gives the specific gravity. Specific gravity is easy to measure in the laboratory but is less useful for mineral identification in the field than other more easily observed properties, except in a few rare cases such as the very dense galena or native gold. The high density of these minerals gives rise to a qualitative property called “heft.” Experienced geologists can roughly assess specific gravity by heft, a subjective quality of how heavy the specimen feels in one’s hand relative to its size.
A simple test for identifying calcite and dolomite is to drop a bit of dilute hydrochloric acid (10-15% HCl) on the specimen. If the acid drop effervesces or fizzes on the surface of the rock, the specimen is calcite. If it does not, the specimen is scratched to produce a small amount of powder and test with acid again. If the acid drop fizzes slowly on the powdered mineral, the specimen is dolomite. The difference between these two minerals can be seen in the video. Geologists who work with carbonate rocks carry a small dropper bottle of dilute HCl in their field kit. Vinegar, which contains acetic acid, can be used for this test and is used to distinguish non-calcite fossils from limestone. While acidic, vinegar produces less of a fizzing reaction because acetic acid is a weaker acid.
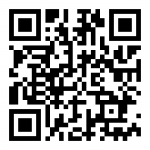
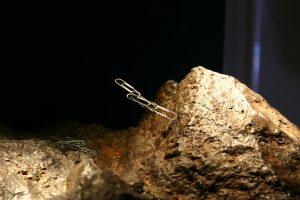
Some iron-oxide minerals are magnetic and are attracted to magnets. A common name for a naturally magnetic iron oxide is lodestone. Others include magnetite (Fe3O4) and ilmenite (FeTiO3). Magnetite is strongly attracted to magnets and can be magnetized. Ilmenite and some types of hematite are weakly magnetic.
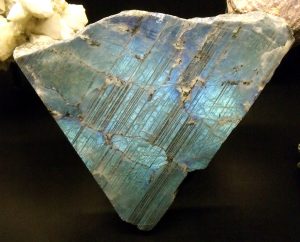
Some minerals and mineraloids scatter light via a phenomenon called iridescence. This property occurs in labradorite (a variety of plagioclase) and opal. It is also seen in biologically created substances like pearls and seashells. Cut diamonds show iridescence and the jeweler’s diamond cut is designed to maximize this property.
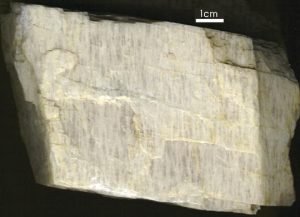
Striations on mineral cleavage faces are an optical property that can be used to separate plagioclase feldspar from potassium feldspar (K-spar). A process called twinning creates parallel zones in the crystal that are repeating mirror images. The actual cleavage angle in plagioclase is slightly different than 90o and the alternating mirror images in these twinned zones produce a series of parallel lines on one of plagioclase’s two cleavage faces. Light reflects off these twinned lines at slightly different angles which then appear as light and dark lines called striations on the cleavage surface. Potassium feldspar does not exhibit twinning or striations but may show linear features called exsolution lamellae, also known as perthitic lineation or simply perthite. Because sodium and potassium do not fit into the same feldspar crystal structure, the lines are created by small amounts of sodium feldspar (albite) separating from the dominant potassium feldspar (K-spar) within the crystal structure. The two different feldspars crystallize out into roughly parallel zones within the crystal, which are seen as these linear markings.
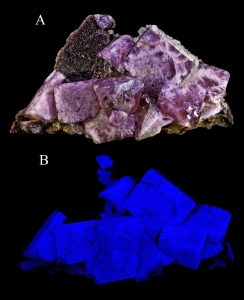
One of the most interesting special mineral properties is fluorescence. Certain minerals, or trace elements within them, give off visible light when exposed to ultraviolet radiation or black light. Many mineral exhibits have a fluorescence room equipped with black lights so this property can be observed. An even rarer optical property is phosphorescence. Phosphorescent minerals absorb light and then slowly release it, much like a glow-in-the-dark sticker.
Take this quiz to check your comprehension of this section.
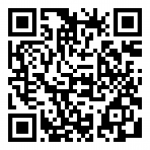
Summary
Minerals are the building blocks of rocks and essential to understanding geology. Mineral properties are determined by their atomic bonds. Most minerals begin in a fluid, and either crystallize out of cooling magma or precipitate as ions and molecules out of a saturated solution. The silicates are largest group of minerals on Earth, by number of varieties and relative quantity, making up a large portion of the crust and mantle. Based on the silicon-oxygen tetrahedra, the crystal structure of silicates reflects the fact that silicon and oxygen are the top two of Earth’s most abundant elements. Non-silicate minerals are also economically important, and providing many types of construction and manufacturing materials. Minerals are identified by their unique physical properties, including luster, color, streak, hardness, crystal habit, fracture, cleavage, and special properties.
Take this quiz to check your comprehension of this Chapter.
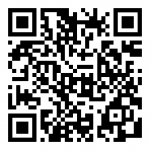
References
- Clarke, F.W.H.S.W., 1927, The Composition of the Earth’s Crust: Professional Paper, United States Geological Survey, Professional Paper.
- Gordon, L.M., and Joester, D., 2011, Nanoscale chemical tomography of buried organic-inorganic interfaces in the chiton tooth: Nature, v. 469, no. 7329, p. 194–197.
- Hans Wedepohl, K., 1995, The composition of the continental crust: Geochim. Cosmochim. Acta, v. 59, no. 7, p. 1217–1232.
- Lambeck, K., 1986, Planetary evolution: banded iron formations: v. 320, no. 6063, p. 574–574.
- metallic bond | chemistry.
- Scerri, E.R., 2007, The Periodic Table: Its Story and Its Significance: Oxford University Press, USA.
- Thomson, J.J., 1897, XL. Cathode Rays: Philosophical Magazine Series 5, v. 44, no. 269, p. 293–316.
- Trenn, T.J., Geiger, H., Marsden, E., and Rutherford, E., 1974, The Geiger-Marsden Scattering Results and Rutherford’s Atom, July 1912 to July 1913: The Shifting Significance of Scientific Evidence: Isis, v. 65, no. 1, p. 74–82.
A type of lamination that is cyclical, perhaps seasonal or diurnal.
A proven commodity of profitable material that could be mined.
The measure of the amount of circular or elliptical nature of the Earth's orbit.
QR Code generated with QRCode Monkey. All generated QR Codes are 100% free and can be used for whatever you want. This includes all commercial purposes.
A specific layer of rock with identifiable properties.
QR Code generated with QRCode Monkey. All generated QR Codes are 100% free and can be used for whatever you want. This includes all commercial purposes.
https://waterdata.usgs.gov/nwis/dv/?ts_id=143976&format=img_default&site_no=404356111503901&set_arithscale_y=on&begin_date=19750718&end_date=19890930
QR Code generated with QRCode Monkey. All generated QR Codes are 100% free and can be used for whatever you want. This includes all commercial purposes.
The study of rock layers and their relationships to each other within a specific area.
Thin (less than 1 cm) beds of rock.
A specific type of sedimentary structure (ripples, plane beds, etc.) linked to a specific flow regime.
A sequence of layers in which the sediment changes linearly in size, either getting coarser or finer.
Predictable sequence of fining upward sediments, caused by turbidity flows.
By Hermann Luyken (Own work) [CC0], via Wikimedia Commons
A qualitative measure of the speed of a fluid flow, with different amounts of flow corresponding to different sedimentary structures, called bedforms. Typically, it is split into upper and lower flow regimes, with upper being a more rapid flow.
A specific layer of rock formed by flowing fluid, either in the lowest part of the lower flow regime or lower part of the upper flow regime.
By דקי [CC BY-SA 3.0 or GFDL], from Wikimedia Commons
Smooth surface carved in harder rocks by glacial action.
(Source: National Park Service modified after Garber et al. 1989)
Part of a glacier which has a net loss of material over the course of a year.
Pieces of mudcracks that are incorporated into a sedimentary rock.
Dunes that are much longer than wide, forming from wind that varies in two opposite directions.
Lake that forms in a kettle.
Ridge of sediment that forms under a glacier by meltwater which forms a river.
Large sediment (e.g. boulder) carried and then dropped by a glacier.
An alpine glacier that fills a mountain valley.
Similar to dunes, in that they are ridges of sand that form perpendicular to flow, but internally, the sediments dip up stream. Forms in the upper part of the upper flow regime.
Ridges of sediment that form perpendicular to flow in the lower part of the lower flow regime.
Photo credit to Louis J. Maher, Jr.
A large pile of sediment, deposited perpendicular to flow. Internal bedding in dunes dips toward flow direction (i.e. cross bedding). Formed in the upper part of the lower flow regime.
The measure of degrees north or south from the equator, which has a latitude of 0 degrees. The Earth's north and south poles have latitudes of 90 degrees north and south, respectively.
A sedimentary structure that forms in the lower flow regime, where ridges of sediment form perpendicular to flow direction, but within the ridges, sediment layers and dips toward flow direction. Found in ripples and dunes. Can be tabular, sinuous, or trough shaped.
Sedimentary layering disturbed by movement of organisms.
QR Code generated with QRCode Monkey. All generated QR Codes are 100% free and can be used for whatever you want. This includes all commercial purposes.
A special type of cross bedding that forms when strong storms produce mounds and divots of cross-bedded sand in deeper water.
Polygonal cracking that occurs with shrinking clays. Indicative of mud submerged underwater and then exposed to air.
By דקי [CC BY-SA 3.0 or GFDL], from Wikimedia Commons
By דקי [CC BY-SA 3.0 or GFDL], from Wikimedia Commons
By Ji-ElleIt feels nice and warmIt feels like a ________ (Own work) [CC BY-SA 3.0], via Wikimedia Commons
By ThaLibster [CC BY-SA 4.0], from Wikimedia Commons
Carbonate rock that reacts with hot magmatic fluids, creating concentrated ore deposits, which include copper, iron, zinc, and gold.
Lake that forms next to a glacier because of crustal loading.
Where a dense ocean plate subducts beneath a less dense oceanic plate, causing an island arc to form.
By Pamputt (Own work) [CC BY-SA 4.0], via Wikimedia Commons
USGS, Public domain
This is a copyrighted image from the CAMECA Archives
Reproduction is authorized, under the terms of the GNU Free Documentation License.
NASA, public domain
By Dirk Hünniger; Derivative work in english - Balajijagadesh [CC BY-SA 3.0], via Wikimedia Commons
By Sbyrnes321 (Own work) [Public domain], via Wikimedia Commons
By Inductiveload (Own work) [Public domain], via Wikimedia Commons
Area behind the arc, which can be subject to compressional (causing thrusted mountain belts) or extensional (causing back-arc basins) forces.
Groves scratched in rock by glacial action.
A process where ice from the ends of glaciers falls off into the ocean.
The line between the zone of accumulation and the zone of ablation.
A terminal moraine that forms as a glacier melts.
Photo by Foto Chd (german wikipedia, https://de.wikipedia.org/wiki/Benutzer:Chd), used under the terms of the GNU Free Documentation License
Any downhill movement of material, caused by gravity.
Steep spire carved by several glaciers.
Thick glaciers that cover continents during ice ages.
Place where two plates slide past each other, creating strike slip faults.
By Tim Bertelink (Own work) [CC BY-SA 4.0], via Wikimedia Commons
Slope angle where shear forces and normal forces are equal.
By Peter Kapitola (Own work) [CC BY-SA 2.5], via Wikimedia Commons
by Robert A. Rohde
A rock made primarily of clay.
By Mike Christie (Own work) [CC BY-SA 3.0], via Wikimedia Commons
A piece of foreign rock that has been incorporated into a magma body. This can be a different type of magma, or a mantle xenolith, a rock from the mantle brought up near the surface.