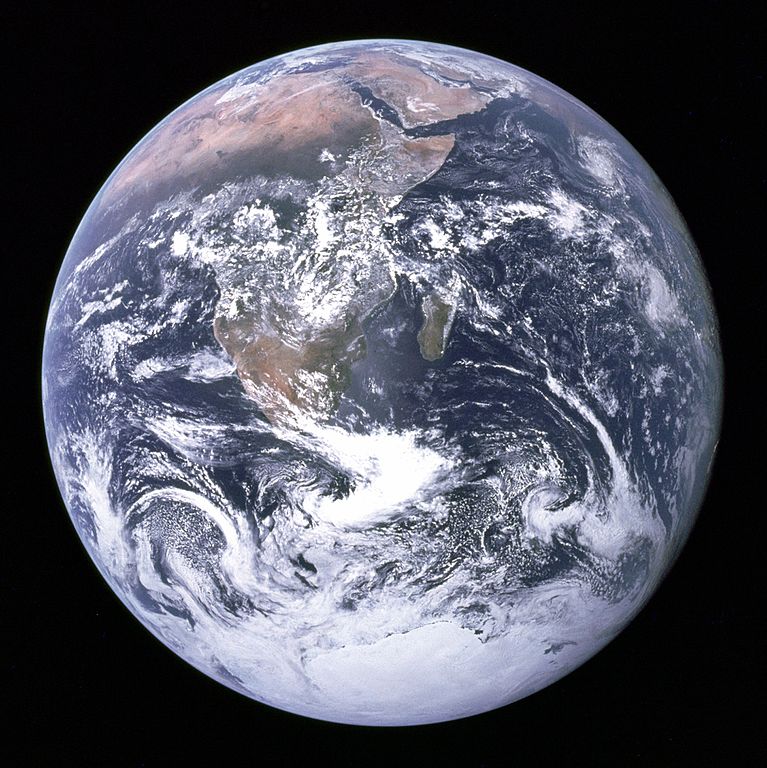
15 Global Climate Change
KEY CONCEPTS
At the end of this chapter, students should be able to:
- Describe the role of greenhouse gases in climate change.
- Describe the sources of greenhouse gases.
- Explain Earth’s energy budget and global temperature changes.
- Explain how positive and negative feedback mechanisms can influence climate.
- Explain how we know about climates in the geologic past.
- Accurately describe which aspects of the environment are changing due to anthropogenic climate change.
- Describe the causes of recent climate change, particularly the role of humans in the overall climate balance
This chapter describes the Earth systems involved in climate change, the geologic evidence of past climate changes, and the human role in today’s climate change. In science, a system is a group of interacting objects and processes. Earth System Science is the study of these systems: geosphere—rocks; atmosphere—gasses; hydrosphere—water; cryosphere—ice; and biosphere—living things. Earth science studies these systems and how they interact and change in response to natural cycles and human-driven, or anthropogenic forces. Changes in one Earth system affect other systems.
It is critically important for us to be aware of the geologic context of climate change processes and how these Earth systems interact, first, for us to understand how and why human activities cause present-day climate change and, secondly, to distinguish between natural processes and human processes in the geologic past’s climate record.
A significant part of this chapter introduces and discusses various processes from these Earth systems, how they influence each other, and how they impact global climate. For example, Earth’s temperature and climate largely change based on atmospheric gas composition, ocean circulation, and the land-surface characteristics of rocks, glaciers, and plants.
Also necessary to understanding climate change is to distinguish between climate and weather. Weather is the short-term temperature and precipitation patterns that occur in days and weeks. Climate is the variable range of temperature and precipitation patterns averaged over the long-term for a particular region (see Chapter 13.1). Thus, a single cold winter does not mean that the entire globe is cooling—indeed, the United States’ cold winters of 2013 and 2014 occurred while the rest of the Earth was experiencing record warm-winter temperatures. To avoid these generalizations, many scientists use a 30-year average as a good baseline. Therefore, climate change refers to slow temperature and precipitation changes and trends over the long term for a particular area or the Earth as a whole.
15.1 Earth’s Temperature
Without an atmosphere, Earth would have huge temperature fluctuations between day and night, like the moon. Daytime temperatures would be hundreds of degrees Celsius above normal, and nighttime temperatures would be hundreds of degrees below normal. Because the Moon doesn’t have much of an atmosphere, its daytime temperatures are around 106 °C (224℉) and nighttime temperatures are around -183°C (-298℉). That is an astonishing 272°C (522°F) degree range between the Moon’s light side and dark side. This section describes how Earth’s atmosphere is involved in regulating the Earth’s temperature.
15.1.1 Composition of Atmosphere
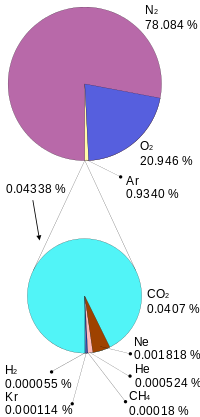
The atmosphere’s composition is a key component in regulating the planet’s temperature. The atmosphere is 78 percent nitrogen (N2), 21 percent oxygen (O2), one percent argon (Ar), and less than one percent trace components, which are all other gases. Trace components include carbon dioxide (CO2), water vapor (H2O), neon, helium, and methane. Water vapor is highly variable, mostly based on region, and composes about one percent of the atmosphere. Trace component gasses include several important greenhouse gases, which are the gases responsible for warming and cooling the plant. On a geologic scale, volcanoes and the weathering process, which bury CO2 in sediments, are the atmosphere’s CO2 sources. Biological processes both add and subtract CO2 from the atmosphere.
Greenhouse gases trap heat in the atmosphere and warm the planet by absorbing some of the longer-wave outgoing infrared radiation that is emitted from Earth, thus keeping heat from being lost to space. More greenhouse gases in the atmosphere absorb more longwave heat and make the planet warmer. Greenhouse gasses have little effect on shorter-wave incoming solar radiation.
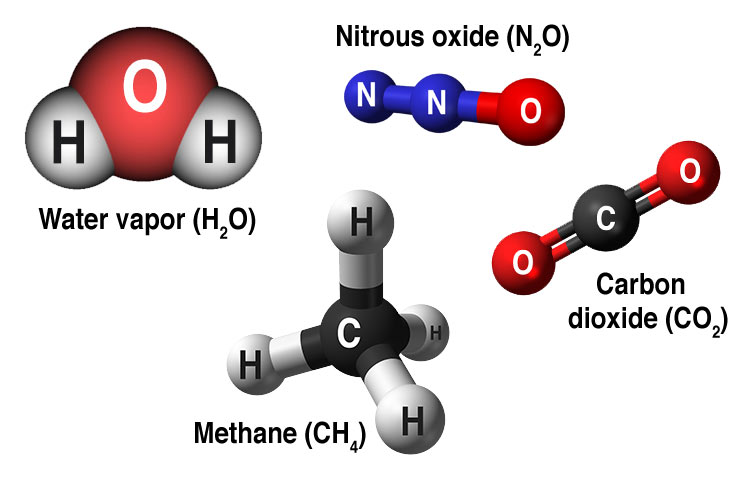
The most common greenhouse gases are water vapor (H2O), carbon dioxide (CO2), methane (CH4), and nitrous oxide (N2O). Water vapor is the most abundant greenhouse gas, but its atmospheric abundance does not change much over time. Carbon dioxide is much less abundant than water vapor, but carbon dioxide is being added to the atmosphere by human activities such as burning fossil fuels, land-use changes, and deforestation. Further, natural processes such as volcanic eruptions add carbon dioxide, but at an insignificant rate compared to human-caused contributions.
There are two important reasons why carbon dioxide is the most important greenhouse gas. First, carbon dioxide stays in the atmosphere and does not go away for hundreds of years. Second, most of the additional carbon dioxide is “fossil” in origin, which means that it is released by burning fossil fuels. For example, coal and petroleum are fossil fuels. Coal and oil are made from long-dead plant material, which was originally created by photosynthesis millions of years ago and stored in the ground. Photosynthesis takes sunlight plus carbon dioxide and creates the substances of plants. This transformation occurs over millions of years as a slow process, accumulating fossil carbon in rocks and sediments. So, when we burn coal and oil, we instantaneously release the stored solar energy and fossil carbon dioxide that took millions of years to accumulate in the first place. The rate of release is critical to comprehend current climate change.
15.1.2 Carbon Cycle
Critical to understanding global climate change is to understand the carbon cycle and how Earth’s own carbon-balancing system is being rapidly thrown off balance by human-driven activities. Earth has two important carbon cycles: the biological and the geological. In the biological cycle, living organisms—mostly plants—consume carbon dioxide from the atmosphere to make their tissues and substances through photosynthesis. Then, after the organisms die, and when they decay over years or decades, that carbon is released back into the atmosphere. The following is the general equation for photosynthesis.
CO2 + H2O + sunlight → sugars + O2
In the geological carbon cycle, a portion of the biological-cycle carbon becomes part of the geological carbon cycle: plant materials into coal and petroleum, tiny fragments and molecules into organic-rich shale, and the carbonate bearing calcareous shells and other parts of marine organisms into limestone. Such materials become buried and become part of the slow geologic formation of coal and other sedimentary materials. This cycle actually involves most of Earth’s carbon and operates very slowly.
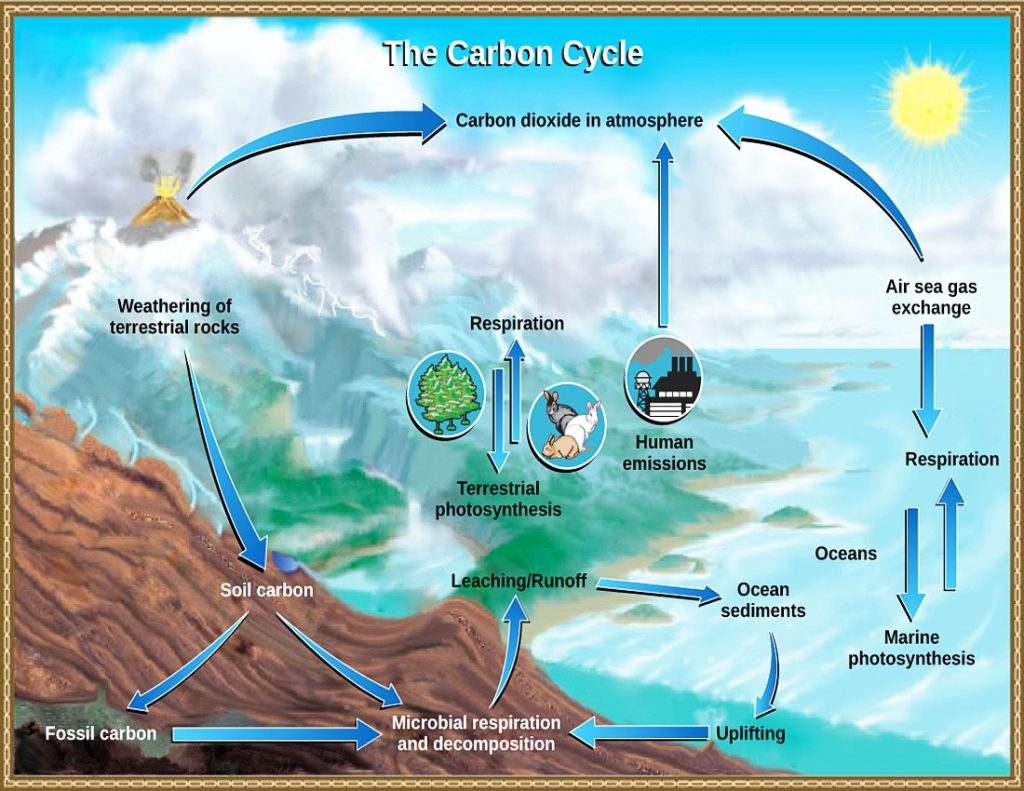
The following are geological carbon-cycle storage reservoirs:
- Organic matter from plants is stored in peat, coal, and permafrost for thousands to millions of years.
- Silicate–mineral weathering converts atmospheric carbon dioxide to dissolved bicarbonate, which is stored in the oceans for thousands to tens of thousands of years.
- Marine organisms convert dissolved bicarbonate to forms of calcite, which is stored in carbonate rocks for tens to hundreds of millions of years.
- Carbon compounds are directly stored in sediments for tens to hundreds of millions of years; some end up in petroleum deposits.
- Carbon-bearing sediments are transferred by subduction to the mantle, where the carbon may be stored for tens of millions to billions of years.
- Carbon dioxide from within the Earth is released back to the atmosphere during volcanic eruptions, where it is stored for years to decades.
During much of Earth’s history, the geological carbon cycle has been balanced by volcanos releasing carbon at approximately the same rate that carbon is stored by the other processes. Under these conditions, Earth’s climate has remained relatively stable. However, in Earth’s history, there have been times when that balance has been upset. This can happen during prolonged stretches of above-average volcanic activity. One example is the Siberian Traps eruption around 250 million years ago, which contributed to strong climate warming over a few million years.
A carbon imbalance is also associated with significant mountain-building events. For example, the Himalayan Range has been forming for about 40 million years, and over that time — and still today — the rate of weathering on Earth has been enhanced because those mountains are so huge and the range is so extensive that they present a greater surface area on which weathering takes place. The weathering of these rocks — most importantly the hydrolysis of feldspar — has resulted in consumption of atmospheric carbon dioxide and transfer of the carbon to the oceans and to ocean-floor carbonate-rich sediments. The steady drop in carbon dioxide levels over the past 40 million years, which contributed to the Pliocene-Pleistocene glaciations, is partly attributable to the formation of the Himalayan Range.
Another, nongeological form of carbon-cycle imbalance is happening today on a very rapid time scale. In just a few decades, humans have extracted volumes of fossil fuels, such as coal, oil, and gas, which were stored in rocks over the past several hundred million years, and converted these fuels to energy and carbon dioxide. By doing so, we are changing the climate faster than has ever happened in the past. Remember, carbon dioxide stays in the atmosphere and does not go away for hundreds of years. The more greenhouse gases in the atmosphere, the more heat is trapped and the warmer the planet becomes.
15.1.3 Greenhouse Effect
The greenhouse effect is the reason our global temperature is rising, but it’s important to understand what this effect is and how it occurs. The greenhouse effect occurs because greenhouse gases are present in the atmosphere. The greenhouse effect is named after a similar process that warms a greenhouse or a car on a hot summer day. Sunlight passes through the glass of the greenhouse or car, reaches the interior, and changes into heat. The heat radiates upward and gets trapped by the glass windows. The greenhouse effect for the Earth can be explained in three steps.
Step 1: Solar radiation from the sun is composed of mostly ultraviolet (UV), visible light, and infrared (IR) radiation. Components of solar radiation include parts with a shorter wavelength than visible light, like ultraviolet light, and parts of the spectrum with longer wavelengths, like IR and others. Some of the radiation gets absorbed, scattered, or reflected by the atmospheric gases but about half of the solar radiation eventually reaches the Earth’s surface.
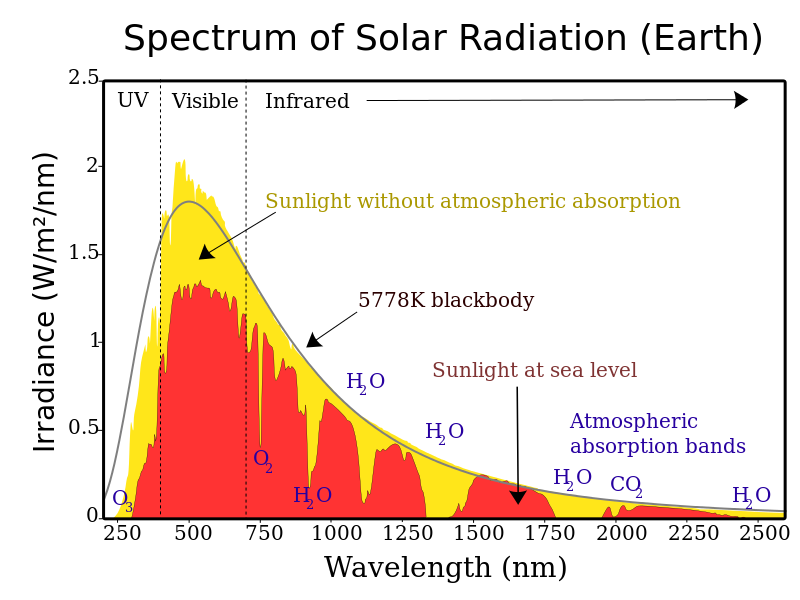
Step 2: The visible, UV, and IR radiation, that reaches the surface converts to heat energy. Most students have experienced sunlight warming a surface such as pavement, a patio, or deck. When this occurs, the warmer surface then emits thermal radiation, which is a type of IR radiation. So, there is a conversion from visible, UV, and IR to just thermal IR. This thermal IR is what we experience as heat. If you have ever felt heat radiating from a fire or a hot stove top, then you have experienced thermal IR.
Step 3: Thermal IR radiates from the earth’s surface back into the atmosphere. But since it is thermal IR instead of UV, visible, or regular IR, this thermal IR gets trapped by greenhouse gases. In other words, the sun’s energy leaves the Earth at a different wavelength than it enters, so the sun’s energy is not absorbed in the lower atmosphere when energy is coming in, but rather when the energy is going out. The gases that are mostly responsible for this energy blocking on Earth include carbon dioxide, water vapor, methane, and nitrous oxide. More greenhouse gases in the atmosphere results in more thermal IR being trapped. Explore this external link to an interactive animation on the greenhouse effect from the National Academy of Sciences.
15.1.4 Earth’s Energy Budget
The solar radiation that reaches Earth is relatively uniform over time. Earth is warmed, and energy or heat radiates from the Earth’s surface and lower atmosphere back to space. This flow of incoming and outgoing energy is Earth’s energy budget. For Earth’s temperature to be stable over long stretches of time, incoming energy and outgoing energy have to be equal on average so that the energy budget at the top of the atmosphere balances. About 29 percent of the incoming solar energy arriving at the top of the atmosphere is reflected back to space by clouds, atmospheric particles, or reflective ground surfaces like sea ice and snow. About 23 percent of incoming solar energy is absorbed in the atmosphere by water vapor, dust, and ozone. The remaining 48 percent passes through the atmosphere and is absorbed at the surface. Thus, about 71 percent of the total incoming solar energy is absorbed by the Earth system.
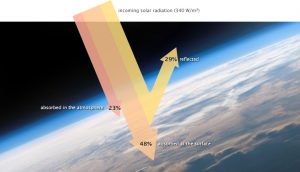
When this energy reaches Earth, the atoms and molecules that makeup the atmosphere and surface absorb the energy, and Earth’s temperature increases. If this material only absorbed energy, then the temperature of the Earth would continue to increase and eventually overheat. For example, if you continuously run a faucet in a stopped-up sink, the water level rises and eventually overflows. However, temperature does not infinitely rise because the Earth is not just absorbing sunlight; it is also radiating thermal energy or heat back into the atmosphere. If the temperature of the Earth rises, the planet emits an increasing amount of heat to space, and this is the primary mechanism that prevents Earth from continually heating.
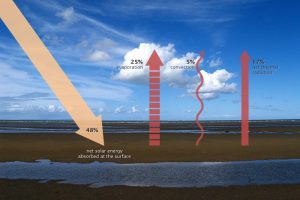
Some of the thermal infrared heat radiating from the surface is absorbed and trapped by greenhouse gasses in the atmosphere, which act like a giant canopy over Earth. The more greenhouse gases in the atmosphere, the more outgoing heat Earth retains, and the less thermal infrared heat dissipates to space.
Factors that can affect the Earth’s energy budget are not limited to greenhouse gases. Increasing solar energy can increase the energy Earth receives. However, these increases are very small over time. In addition, land and water will absorb more sunlight when there is less ice and snow to reflect the sunlight back to the atmosphere. For example, the ice covering the Arctic Sea reflects sunlight back to the atmosphere; this reflectivity is called albedo. Furthermore, aerosols (dust particles) produced from burning coal, diesel engines, and volcanic eruptions can reflect incoming solar radiation and actually cool the planet. While the effect of anthropogenic aerosols on the climate’s system is weak, the effect of human-produced greenhouse gases is not weak. Thus, the net effect of human activity is warming due to more anthropogenic greenhouse gases associated with fossil fuel combustion.
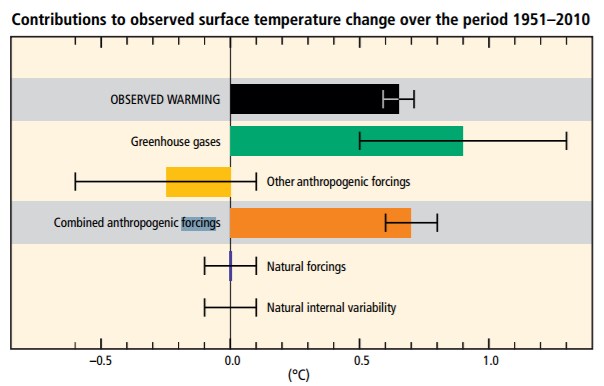
An effect that changes the planet can trigger feedback mechanisms that amplify or suppress the original effect. A positive feedback mechanism occurs when the output or effect of a process enhances the original stimulus or cause. Thus, it increases the ongoing effect. For example, the loss of sea ice at the North Pole makes that area less reflective, reducing albedo. This allows the surface air and ocean to absorb more energy in an area that was once covered by sea ice. Another example is melting permafrost. Permafrost is permanently frozen soil located in the high latitudes, mostly in the Northern Hemisphere. As the climate warms, more permafrost thaws, and the thick deposits of organic matter are exposed to oxygen and begin to decay. This oxidation process releases carbon dioxide and methane, which in turn causes more warming, which melts more permafrost, and so on and on.
A negative feedback mechanism occurs when the output or effect reduces the original stimulus or cause. For example, in the short term, more carbon dioxide (CO2) is expected to cause forest canopies to grow, which absorb more CO2. Another example for the long term is that increased carbon dioxide in the atmosphere will cause more carbonic acid and chemical weathering, which results in transporting dissolved bicarbonate and other ions to the oceans, which are then stored in sediment.
Global warming is evidence that Earth’s energy budget is not balanced. Positive effects on Earth’s temperature are now greater than negative effects.
Take this quiz to check your comprehension of this section.
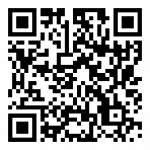
15.2 Evidence of Recent Climate Change
While climate has changed often in the past due to natural causes (see chapter 14.5.1 and chapter 15.3), the scientific consensus is that human activity is causing current very rapid climate change. While this seems like a new idea, it was suggested more than 75 years ago. This section describes the evidence of what most scientists agree is anthropogenic or human-caused climate change. For more information, watch this six-minute video on climate change by two professors at the North Carolina State University.
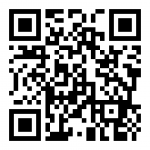
15.2.1 Global Temperature Rise
The land-ocean temperature index, 1880 to present, compared to a base reference time of 1951-1980, shows ocean temperatures steadily rising. The solid black line is the global annual mean, and the solid red line is the five-year Lowess smoothing. The blue uncertainty bars (95 percent confidence limit) account only for incomplete spatial sampling.
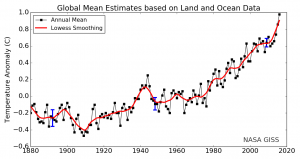
Since 1880, Earth’s surface-temperature average has trended upward with most of that warming occurring since 1970 (see this NASA animation). Surface temperatures include both land and ocean because water absorbs much additional trapped heat. Changes in land-surface or ocean-surface temperatures compared to a reference period from 1951 to 1980, where the long-term average remained relatively constant, are called temperature anomalies. A temperature anomaly thus represents the difference between the measured temperature and the average value during the reference period. Climate scientists calculate long-term average temperatures over thirty years or more which identified the reference period from 1951 to 1980. Another common range is a century, for example, 1900-2000. Therefore, an anomaly of 1.25 ℃ (34.3°F) for 2015 means that the average temperature for 2015 was 1.25 ℃ (34.3°F) greater than the 1900-2000 average. In 1950, the temperature anomaly was -0.28 ℃ (31.5°F), so this is -0.28 ℃ (31.5°F) lower than the 1900-2000 average. These temperatures are annual average measured surface temperatures.
This video figure of temperature anomalies shows worldwide temperature changes since 1880. The more blue, the cooler; the more yellow and red, the warmer.
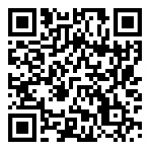
In addition to average land-surface temperatures rising, the ocean has absorbed much heat. Because oceans cover about 70 percent of the Earth’s surface and have such a high specific heat value, they provide a large opportunity to absorb energy. The ocean has been absorbing about 80 to 90 percent of human activities’ additional heat. As a result, temperature in the ocean’s top 701.4 m (2,300 ft) has increased by -17.6°C (0.3℉) since 1969 (watch this 3 minute video by NASA JPL on the ocean’s heat capacity). The reason the ocean has warmed less than the atmosphere, while still taking on most of the heat, is due to water’s very high specific heat, which means that water can absorb a lot of heat energy with a small temperature increase. In contrast, the lower specific heat of the atmosphere means it has a higher temperature increase as it absorbs less heat energy.
Some scientists suggest that anthropogenic greenhouse gases do not cause global warming because between 1998 and 2013, Earth’s surface temperatures did not increase much, despite greenhouse gas concentrations continuing to increase. However, since the oceans are absorbing most of the heat, decade-scale circulation changes in the ocean, similar to La Niña, push warmer water deeper under the surface. Once the ocean’s absorption and circulation is accounted for, and this heat is added back into surface temperatures, then temperature increases become apparent, as shown in the figure. Also, the ocean’s heat storage is temporary, as reflected in the record-breaking warm years of 2014-2016. Indeed, with this temporary ocean-storage effect, the twenty-first century’s first 15 of its 16 years were the hottest in recorded history.
15.2.2 Carbon Dioxide
Anthropogenic greenhouse gases, mostly carbon dioxide (CO2), have increased since the industrial revolution when humans dramatically increased burning fossil fuels. These levels are unprecedented in the last 800,000-year Earth history as recorded in geologic sources such as ice cores. Carbon dioxide has increased by 40 percent since 1750, and the rate of increase has been the fastest during the last decade. For example, since 1750, 20409 tonnes (2040 gigatons) of CO2 have been added to the atmosphere; about 40 percent has remained in the atmosphere while the remaining 60 percent has been absorbed into the land by plants and soil or into the oceans. Indeed, during the lifetime of most young adults, the total atmospheric CO2 has increased by 50 ppm, or 15 percent.
Charles Keeling, an oceanographer with Scripps Institution of Oceanography in San Diego, California, was the first person to regularly measure atmospheric CO2. Using his methods, scientists at the Mauna Loa Observatory, Hawaii, have constantly measured atmospheric CO2 since 1957. NASA regularly publishes these measurements at https://scripps.ucsd.edu/programs/keelingcurve/. Go there now to see the very latest measurement. Keeling’s measured values have been posted in a curve of increasing values, called the Keeling Curve. This curve varies up and down in a regular annual cycle, from summer when the plants in the Northern Hemisphere are using CO2 to winter when the plants are dormant. But the curve shows a steady CO2 increase over the past several decades. This curve increases exponentially, not linearly, showing that the rate of CO2 increase is itself increasing!
The following Atmospheric CO2 video shows how atmospheric CO2 has varied recently and over the last 800,000 years, as determined by an increasing number of CO2 monitoring stations as shown on the insert map. It is also instructive to watch the video’s Keeling portion of how CO2 varies by latitude. This shows that most human CO2 sources are in the Northern Hemisphere where most of the land is and where most of the developed nations are.
15.2.3 Melting Glaciers and Shrinking Sea Ice
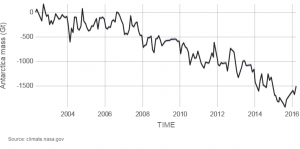
Glaciers are large ice accumulations that exist year round on the land’s surface. In contrast, icebergs are masses of floating sea ice, although they may have had their origin in glaciers (see Chapter 14). Alpine glaciers, ice sheets, and sea ice are all melting. Explore melting glaciers at NASA’s interactive Global Ice Viewer). Satellites have recorded that Antarctica is melting at 1189 tonnes (118 gigatons) per year, and Greenland is melting at 2819 tonnes (281 gigatons) per year; 1 metric tonne is 1000 kilograms (1 gigaton is over 2 trillion pounds). Almost all major alpine glaciers are shrinking, deflating, and retreating. The ice-mass loss rate is unprecedented—never observed before—since the 1940’s when quality records for glaciers began.
Before anthropogenic warming, glacial activity was variable with some retreating and some advancing. Now, spring snow cover is decreasing, and sea ice is shrinking. Most sea ice is at the North Pole, which is only occupied by the Arctic Ocean and sea ice. The NOAA animation shows how perennial sea ice has declined from 1987 to 2015. The oldest ice is white, and the youngest, seasonal ice is dark blue. The amount of old ice has declined from 20 percent in 1985 to 3 percent in 2015.
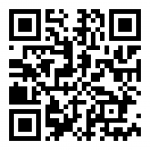
15.2.4 Rising Sea-Level
Sea level is rising 3.4 millimeters (0.13 inches) per year and has risen 0.19 meters (7.4 inches) from 1901 to 2010. This is thought largely to be from both glaciers melting and thermal expansion of sea water. Thermal expansion means that as objects such as solids, liquids, and gases heat up, they expand in volume.
Classic video demonstration (30 second) on thermal expansion with brass ball and ring (North Carolina School of Science and Mathematics).
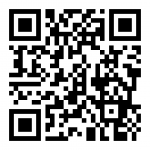
15.2.5 Ocean Acidification
Since 1750, about 40 percent of new anthropogenic carbon dioxide has remained in the atmosphere. The remaining 60 percent gets absorbed by the ocean and vegetation. The ocean has absorbed about 30 percent of that carbon dioxide. When carbon dioxide gets absorbed in the ocean, it creates carbonic acid. This makes the ocean more acidic, which then has an impact on marine organisms that secrete calcium carbonate shells. Recall that hydrochloric acid reacts by effervescing with limestone rock made of calcite, which is calcium carbonate. A more acidic ocean is associated with climate change and is linked to some sea snails (pteropods) and small protozoan zooplanktons’ (foraminifera) thinning carbonate shells and to ocean coral reefs’ declining growth rates. Small animals like protozoan zooplankton are an important component at the base of the marine ecosystem. Acidification combined with warmer temperature and lower oxygen levels is expected to have severe impacts on marine ecosystems and human-harvested fisheries, possibly affecting our ocean-derived food sources.
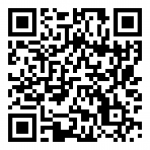
15.2.6 Extreme Weather Events
Extreme weather events such as hurricanes, precipitation, and heatwaves are increasing and becoming more intense. Since the 1980’s, hurricanes, which are generated from warm ocean water, have increased in frequency, intensity, and duration and are likely connected to a warmer climate. Since 1910, average precipitation has increased by 10 percent in the contiguous United States, and much of this increase is associated with heavy precipitation events. However, the distribution is not even, and more precipitation is projected for the northern United States while less precipitation is projected for the already dry southwest. Also, heatwaves have increased, and rising temperatures are already affecting crop yields in northern latitudes. Increased heat allows for greater moisture capacity in the atmosphere, increasing the potential for more extreme events.
Take this quiz to check your comprehension of this section.
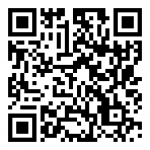
15.3 Prehistoric Climate Change
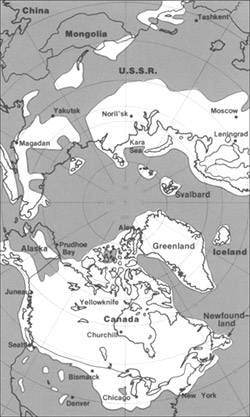
Over Earth’s history, the climate has changed a lot. For example, during the Mesozoic Era, the Age of Dinosaurs, the climate was much warmer, and carbon dioxide was abundant in the atmosphere. However, throughout the Cenozoic Era, 65 million years ago to today, the climate has been gradually cooling. This section summarizes some of these major past climate changes.
15.3.1 Past Glaciations
Through geologic history, climate has changed slowly over millions of years. Before the most recent Pliocene-Quaternary glaciation, there were other major glaciations. The oldest, known as the Huronian, occurred toward the end of the Archean Eon-early Proterozoic Eon, about 2.5 billion years ago. The event of that time, the Great Oxygenation Event, was a major happening (see Chapter 8) most commonly associated with causing that glaciation. The increased oxygen is thought to have reacted with the potent greenhouse gas methane, causing cooling.
The end of the Proterozoic Eon, about 700 million years ago, had other glaciations. These ancient Precambrian glaciations are included in the Snowball Earth hypothesis. Widespread global rock sequences from these ancient times contain evidence that glaciers existed even in low-latitudes. Two examples are limestone rock—usually formed in tropical marine environments—and glacial deposits—usually formed in cold climates—have been found together from this time in many regions around the world. One example is in Utah. Evidence of continental glaciation is seen in interbedded limestone and glacial deposits (diamictites) on Antelope Island in the Great Salt Lake.
The controversial Snowball Earth hypothesis suggests that a runaway albedo effect—where ice and snow reflect solar radiation and increasingly spread from polar regions toward the equator—caused land and ocean surfaces to completely freeze and biological activity to collapse. Thinking is that because carbon dioxide could not enter the then-frozen ocean, the ice covering Earth could only melt when volcanoes emitted high enough carbon dioxide into the atmosphere to cause greenhouse heating. Some studies estimate that because of the frozen ocean surface, carbon dioxide 350 times higher than today’s concentration was required. Because biological activity did survive, the complete freezing and its extent in the snowball earth hypothesis are controversial. A competing hypothesis is the Slushball Earth hypothesis in which some regions of the equatorial ocean remained open. Differing scientific conclusions about the stability of Earth’s magnetic poles, impacts on ancient rock evidence from subsequent metamorphism, and alternate interpretations of existing evidence keep the idea of Snowball Earth controversial.
Glaciations also occurred in the Paleozoic Era, notably the Andean-Saharan glaciation in the late Ordovician, about 440–460 million years ago, which coincided with a major extinction event, and the Karoo Ice Age during the Pennsylvanian Period, 323 to 300 million years ago. This glaciation was one of the evidences cited by Wegener for his Continental Drift hypothesis as his proposed Pangea drifted into south polar latitudes. The Karoo glaciation was associated with an increase of oxygen and a subsequent drop in carbon dioxide, most likely produced by the evolution and rise of land plants.
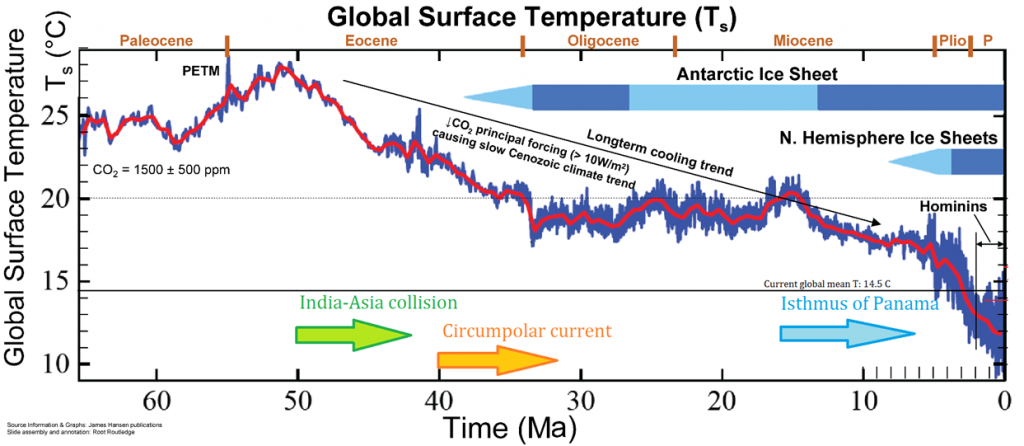
During the Cenozoic Era—the last 65 million years, climate started out warm and gradually cooled to today. This warm time is called the Paleocene-Eocene Thermal Maximum, and Antarctica and Greenland were ice free during this time. Since the Eocene, tectonic events during the Cenozoic Era caused the planet to persistently and significantly cool. For example, the Indian Plate and Asian Plate collided, creating the Himalaya Mountains, which increased the rate of weathering and erosion of silicate minerals, especially feldspar. Increased weathering consumes carbon dioxide from the atmosphere, which reduces the greenhouse effect, resulting in long-term cooling.
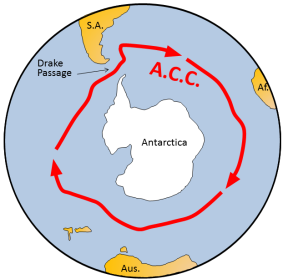
About 40 million years ago, the narrow gap between the South American Plate and the Antarctica Plate widened, which opened the Drake Passage. This opening allowed the water around Antarctica—the Antarctic Circumpolar Current—to flow unrestrictedly west-to-east, which effectively isolated the southern ocean from the warmer waters of the Pacific, Atlantic, and Indian Oceans. The region cooled significantly, and by 35 million years ago, during the Oligocene Epoch, glaciers had started to form on Antarctica.
Around 15 million years ago, subduction-related volcanos between Central and South America created the Isthmus of Panama, which connected North and South America. This prevented water from flowing between the Pacific and Atlantic Oceans and reduced heat transfer from the tropics to the poles. This reduced heat transfer created a cooler Antarctica and larger Antarctic glaciers. As a result, the ice sheet expanded on land and water, increased Earth’s reflectivity and enhanced the albedo effect, which created a positive feedback loop: more reflective glacial ice, more cooling, more ice, more cooling, and so on.
By 5 million years ago, during the Pliocene Epoch, ice sheets had started to grow in North America and northern Europe. The most intense part of the current glaciation is the Pleistocene Epoch’s last 1 million years. The Pleistocene’s temperature varies significantly through a range of almost 10°C (18°F) on time scales of 40,000 to 100,000 years, and ice sheets expand and contract correspondingly. These variations are attributed to subtle changes in Earth’s orbital parameters, called Milankovitch cycles (see Chapter 14). Over the past million years, the glaciation cycles occurred approximately every 100,000 years, with many glacial advances occurring in the last 2 million years (Lisiecki and Raymo, 2005).
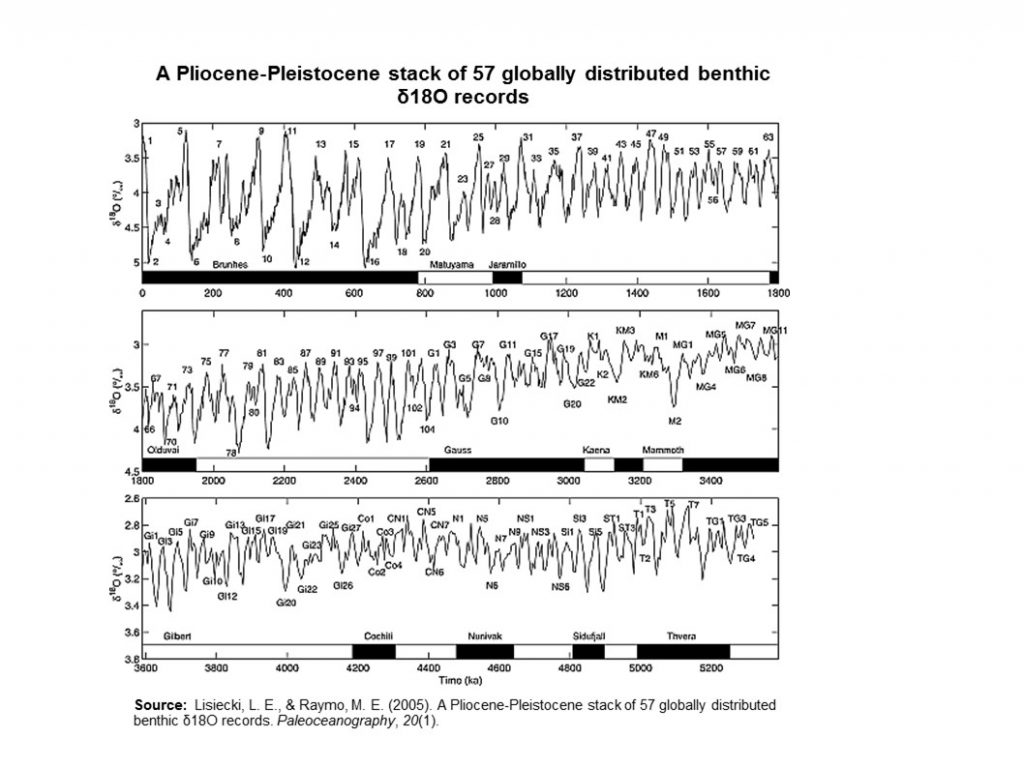
During an ice age, periods of warming climate are called interglacials; during interglacials, very brief periods of even warmer climate are called interstadials. These warming upticks are related to Earth’s climate variations, like Milankovitch cycles, which are changes to the Earth’s orbit that can fluctuate climate (see Chapter 14). In the last 500,000 years, there have been five or six interglacials, with the most recent belonging to our current time, the Holocene Epoch.
The two more recent climate swings, the Younger Dryas and the Holocene Climatic Optimum, demonstrate complex changes. These events are more recent, yet have conflicting information. The Younger Dryas’ cooling is widely recognized in the Northern Hemisphere, though the event’s timing, about 12,000 years ago, does not appear to be equal everywhere. Also, it is difficult to find in the Southern Hemisphere. The Holocene Climatic Optimum is a warming around 6,000 years ago; it was not universally warmer, nor as warm as current warming, and not warm at the same time everywhere.
15.3.2 Proxy Indicators of Past Climates
How do we know about past climates? Geologists use proxy indicators to understand past climate. A proxy indicator is a biological, chemical, or physical signature preserved in the rock, sediment, or ice record that acts like a fingerprint of something in the past. Thus, they are an indirect indicator of climate. An indirect indicator of ancient glaciations from the Proterozoic Eon and Paleozoic Era is the Mineral Fork Formation in Utah, which contains rock formations of glacial sediments such as diamictite (tillite). This dark rock has many fine-grained components plus some large out-sized clasts like a modern glacial till.
Deep-sea sediment is an indirect indicator of climate change during the Cenozoic Era, about the last 65 million years. Researchers from the Ocean Drilling Program, an international research collaboration, collect deep-sea sediment cores that record continuous sediment accumulation. The sediment provides detailed chemical records of stable carbon and oxygen isotopes obtained from deep-sea benthic foraminifera shells that accumulated on the ocean floor over millions of years. The oxygen isotopes are a proxy indicator of deep-sea temperatures and continental ice volume.
Sediment Cores – Stable Oxygen Isotopes
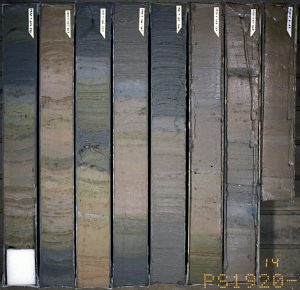
How do oxygen isotopes indicate past climate? The two main stable oxygen isotopes are 16O and 18O. They both occur in water (H2O) and in the calcium carbonate (CaCO3) shells of foraminifera as both of those substances’ oxygen component. The most abundant and lighter isotope is 16O. Since it is lighter, it evaporates more readily from the ocean’s surface as water vapor, which later turns to clouds and precipitation on the ocean and land. This evaporation is enhanced in warmer sea water and slightly increases the concentration of 18O in the surface seawater from which the plankton derives the carbonate for its shells. Thus the ratio of 16O and 18O in the fossilized shells in seafloor sediment is a proxy indicator of the temperature and evaporation of seawater.
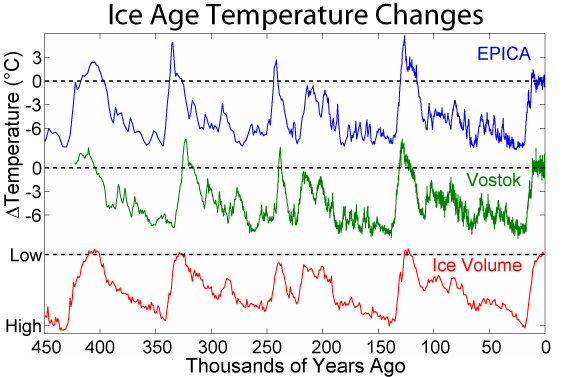
Keep in mind, it is harder to evaporate the heavier water and easier to condense it. As evaporated water vapor drifts toward the poles and tiny droplets form clouds and precipitation, droplets of water with 18O tend to form more readily than droplets of the lighter form and precipitate out, leaving the drifting vapor depleted in 18O. During geologic times when the climate is cooler, more of this lighter precipitation that falls on land is locked in the form of glacial ice. Consider that the giant ice sheets were more than a mile thick and covered a large part of North America during the last ice age only 14,000 years ago. During glaciation, the glaciers thus effectively lock away more 16O, thus the ocean water and foraminifera shells become enriched in 18O. Therefore, the ratio of 18O to 16O (𝛿18O) in calcium carbonate shells of foraminifera is a proxy indicator of past climate. The sediment cores from the Ocean Drilling Program record a continuous accumulation of these fossils in the sediment and provide a record of glacials, interglacials and interstadials.
Sediment Cores – Boron-Isotopes and Acidity
Ocean acidity is affected by carbonic acid and is a proxy for past atmospheric CO2 concentrations. To estimate the ocean’s pH (acidity) over the past 60 million years, researchers collected deep-sea sediment cores and examined the ancient planktonic foraminifera shells’ boron-isotope ratios. Boron has two isotopes: 11B and 10B. In aqueous compounds of boron, the relative abundance of these two isotopes is sensitive to pH (acidity), hence CO2 concentrations. In the early Cenozoic, around 60 million years ago, CO2 concentrations were over 2,000 ppm, higher pH, and started falling around 55 to 40 million years ago, with noticeable drop in pH, indicated by boron isotope ratios. The drop was possibly due to reduced CO2 outgassing from ocean ridges, volcanoes and metamorphic belts, and increased carbon burial due to subduction and the Himalaya Mountains uplift. By the Miocene Epoch, about 24 million years ago, CO2 levels were below 500 ppm, and by 800,000 years ago, CO2 levels didn’t exceed 300 ppm.
Carbon Dioxide Concentrations in Ice Cores
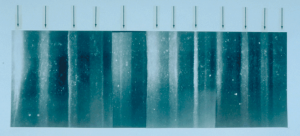
For the recent Pleistocene Epoch’s climate, researchers get a more detailed and direct chemical record of the last 800,000 years by extracting and analyzing ice cores from the Antarctic and Greenland ice sheets. Snow accumulates on these ice sheets and creates yearly layers. Oxygen isotopes are collected from these annual layers, and the ratio of 18O to 16O (𝛿18O) is used to determine temperature as discussed above. In addition, the ice contains small bubbles of atmospheric gas as the snow turns to ice. Analysis of these bubbles reveals the composition of the atmosphere at these previous times.
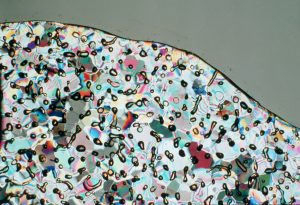
Small pieces of this ice are crushed, and the ancient air is extracted into a mass spectrometer that can detect the ancient atmosphere’s chemistry. Carbon dioxide levels are recreated from these measurements. Over the last 800,000 years, the maximum carbon dioxide concentration during warm times was about 300 parts per million (ppm), and the minimum was about 170 ppm during cold stretches. Currently, the earth’s atmospheric carbon dioxide content is over 410 ppm.
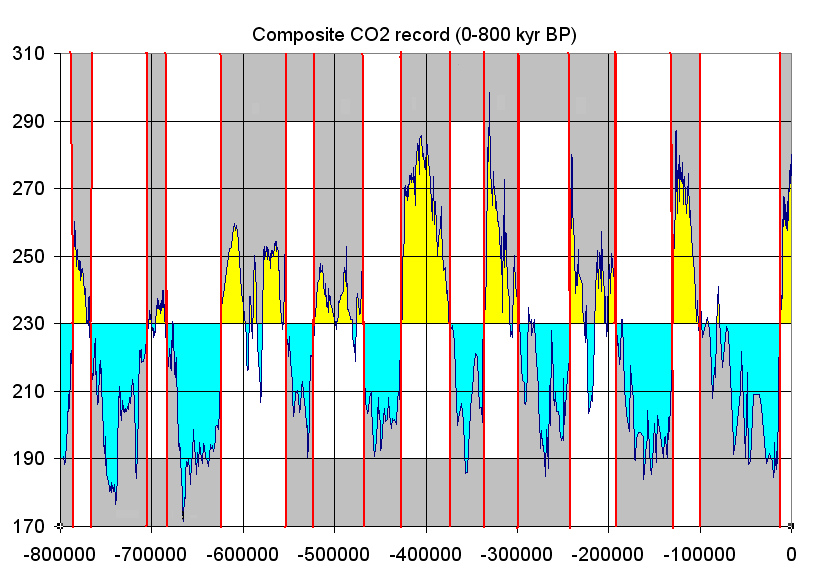
Oceanic Microfossils
Microfossils, like foraminifera, diatoms, and radiolarians can be used as a proxy to interpret past climate record. Different species of microfossils are found in the sediment core’s different layers. Microfossil groups are called assemblages and their composition differs depending on the climatic conditions when they lived. One assemblage consists of species that lived in cooler ocean water, such as in glacial times, and at a different level in the same sediment core, another assemblage consists of species that lived in warmer waters.
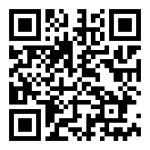
Tree Rings
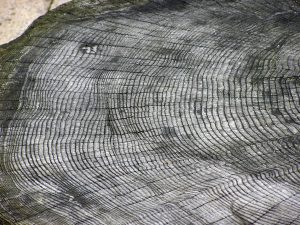
Tree rings, which form every year as a tree grows, are another past climate indicator. Rings that are thicker indicate wetter years, and rings that are thinner and closer together indicate dryer years. Every year, a tree will grow one ring with a light section and a dark section. The rings vary in width. Since trees need much water to survive, narrower rings indicate colder and drier climates. Since some trees are several thousand years old, scientists can use their rings for regional paleoclimatic reconstructions, for example, to reconstruct past temperature, precipitation, vegetation, streamflow, sea-surface temperature, and other climate-dependent conditions. Paleoclimatic study means relating to a distinct past geologic climate. Also, dead trees, such as those found in Puebloan ruins, can be used to extend this proxy indicator by showing long-term droughts in the region and possibly explain why villages were abandoned.
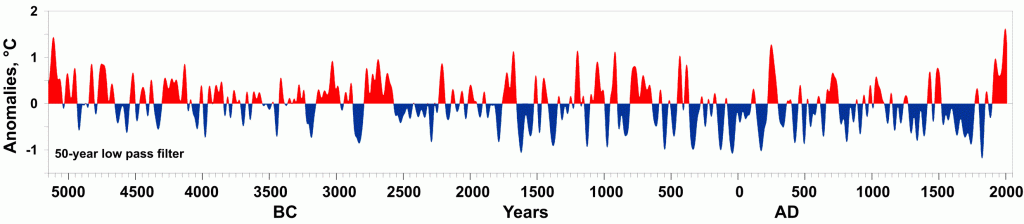
Pollen
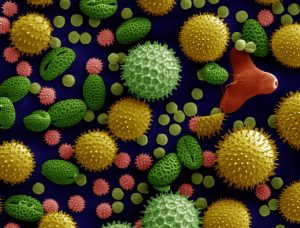
Pollen is also a proxy climate indicator. Flowering plants produce pollen grains. Pollen grains are distinctive when viewed under a microscope. Sometimes, pollen is preserved in lake sediments that accumulate in layers every year. Lake-sediment cores can reveal ancient pollen. Fossil-pollen assemblages are pollen groups from multiple species, such as spruce, pine, and oak. Through time, via the sediment cores and radiometric age-dating techniques, the pollen assemblages change, revealing the plants that lived in the area at the time. Thus, the pollen assemblages are a past climate indicator, since different plants will prefer different climates. For example, in the Pacific Northwest, east of the Cascades in a region close to grassland and forest borders, scientists tracked pollen over the last 125,000 years, covering the last two glaciations. As shown in the figure (Fig. 2 from reference Whitlock and Bartlein 1997), pollen assemblages with more pine tree pollen are found during glaciations and pollen assemblages with less pine tree pollen are found during interglacial times.
Other Proxy Indicators
Paleoclimatologists study many other phenomena to understand past climates, such as human historical accounts, human instrument records from the recent past, lake sediments, cave deposits, and corals.
Take this quiz to check your comprehension of this section.
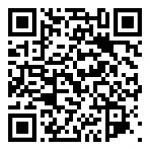
15.4 Anthropogenic Causes of Climate Change
As shown in the previous section, prehistoric climate changes occur slowly over many millions of years. The climate changes observed today are rapid and largely human caused. Evidence shows that climate is changing, but what is causing that change? Since the late 1800s, scientists have suspected that human-produced, i.e. anthropogenic changes in atmospheric greenhouse gases would likely cause climate change because changes in these gases have been the case every time in the geologic past. By the middle 1900s, scientists began conducting systematic measurements, which confirmed that human-produced carbon dioxide was accumulating in the atmosphere and other earth systems, such as forests and oceans. By the end of the 1900s and into the early 2000s, scientists solidified the Theory of Anthropogenic Climate Change when evidence from thousands of ground-based studies and continuous land and ocean satellite measurements mounted, revealing the expected temperature increase. The Theory of Anthropogenic Climate Change is that humans are causing most of the current climate changes by burning fossil fuels such as coal, oil, and natural gas. Theories evolve and transform as new data and new techniques become available, and they represent a particular field’s state of thinking. This section summarizes the scientific consensus of anthropogenic climate change.
15.4.1 Scientific Consensus
The overwhelming majority of climate studies indicate that human activity is causing rapid changes to the climate, which will cause severe environmental damage. There is strong scientific consensus on the issue. Studies published in peer-reviewed scientific journals show that 97 percent of climate scientists agree that climate warming is caused from human activities. There is no alternative explanation for the observed link between human-produced greenhouse gas emissions and changing modern climate. Most leading scientific organizations endorse this position, including the U.S. National Academy of Science, which was established in 1863 by an act of Congress under President Lincoln. Congress charged the National Academy of Science “with providing independent, objective advice to the nation on matters related to science and technology.” Therefore, the National Academy of Science is the leading authority when it comes to policy advice related to scientific issues.
One way we know that the increased greenhouse gas emissions are from human activities is with isotopic fingerprints. For example, fossil fuels, representing plants that lived millions of years ago, have a stable carbon-13 to carbon-12 (13C/12C) ratio that is different from today’s atmospheric stable-carbon ratio (radioactive 14C is unstable). Isotopic carbon signatures have been used to identify anthropogenic carbon in the atmosphere since the 1980s. Isotopic records from the Antarctic Ice Sheet show stable isotopic signatures from ~1000 AD to ~1800 AD and a steady isotopic signature gradually changing since 1800, followed by a more rapid change after 1950 as burning of fossil fuels dilutes the CO2 in the atmosphere. These changes show the atmosphere as having a carbon isotopic signature increasingly more similar to that of fossil fuels.
15.4.2 Anthropogenic Sources of Greenhouse Gases
Anthropogenic emissions of greenhouse gases have increased since pre-industrial times due to global economic growth and population growth. Atmospheric concentrations of the leading greenhouse gas, carbon dioxide, are at unprecedented levels that haven’t been observed in at least the last 800,000 years. Pre-industrial level of carbon dioxide was at about 278 parts per million (ppm). As of 2016, carbon dioxide was, for the first time, above 400 ppm for the entirety of the year. Measurements of atmospheric carbon at the Mauna Loa Carbon Dioxide Observatory show a continuous increase since 1957 when the observatory was established from 315 ppm to over 417 ppm in 2022. The daily reading today can be seen at Daily CO2. Based on the ice core record over the past 800,000 years, carbon dioxide ranged from about 185 ppm during ice ages to 300 ppm during warm times. View the data-accurate NOAA animation below of carbon dioxide trends over the last 800,000 years.
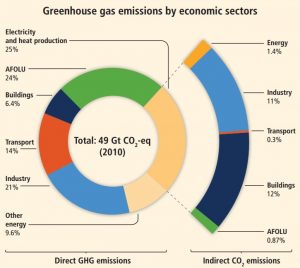
What is the source of these anthropogenic greenhouse gas emissions? Fossil fuel combustion and industrial processes contributed 78 percent of all emissions since 1970. The economic sectors responsible for most of this include electricity and heat production (25 percent); agriculture, forestry, and land use (24 percent); industry (21 percent); transportation, including automobiles (14 percent); other energy production (9.6 percent); and buildings (6.4 percent). More than half of greenhouse gas emissions have occurred in the last 40 years, and 40 percent of these emissions have stayed in the atmosphere. Unfortunately, despite scientific consensus, efforts to mitigate climate change require political action. Despite growing climate change concern, mitigation efforts, legislation, and international agreements have reduced emissions in some places, yet the less developed world’s continual economic growth has increased global greenhouse gas emissions. In fact, the years 2000 to 2010 saw the largest increases since 1970.
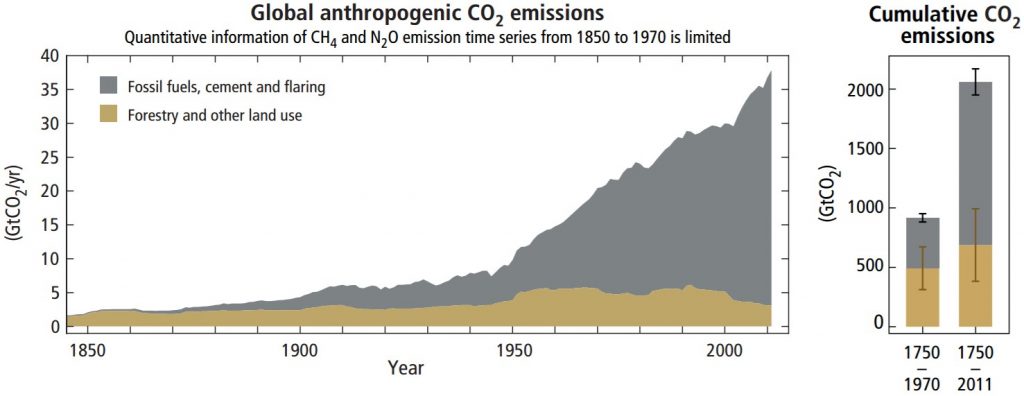
Take this quiz to check your comprehension of this section.
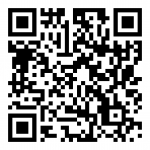
Summary
Included in Earth Science is the study of the system of processes that affect surface environments and atmosphere of the Earth. Recent changes in atmospheric temperature and climate over intervals of decades have been observed. For Earth’s climate to be stable, incoming radiation from the sun and outgoing radiation from the sun-warmed Earth must be in balance. Gases in the atmosphere called greenhouse gases absorb the infrared thermal radiation from the Earth’s surface, trapping that heat and warming the atmosphere, a process called the Greenhouse Effect. Thus the energy budget is not now in balance and the Earth is warming. Human activity produces many greenhouse gases that have accelerated climate change. CO2 from fossil fuel burning is one of the major ones. While atmospheric composition is mostly nitrogen and oxygen, trace components including the greenhouse gases (CO2 and methane are the major ones and there are others) have the greatest effect on global warming.
A number of Positive Feedback Mechanisms, processes whose results reinforce the original process, take place in the Earth system. An example of a PFM of great concern is permafrost melting which causes decay of melting organic material that produces CO2 and methane (both powerful greenhouse gases) that warm the atmosphere and promote more permafrost melting. Two carbon cycles affect Earth’s atmospheric CO2 composition, the biologic carbon cycle and the geologic carbon cycle. In the biologic cycle, organisms (mostly plants and also animals that eat them) remove CO2 from the atmosphere for energy and to build their body tissues and return it to the atmosphere when they die and decay. The biologic cycle is a rapid cycle. In the geologic cycle, some organic matter is preserved in the form of petroleum and coal while more is dissolved in seawater and captured in carbonate sediments, some of which is subducted into the mantle and returned by volcanic activity. The geologic carbon cycle is slow over geologic time.
Measurements of increasing atmospheric temperature have been made since the nineteenth century but the upward temperature trend itself increased in the mid twentieth century showing the current trend is exponential. Because of the high specific heat of water, the oceans have absorbed most of the added heat. That this is temporary storage is revealed by the record-breaking warm years of the recent decade and the increase in intense storms and hurricanes. In 1957 the Mauna Loa CO2 Observatory was established in Hawaii providing constant measurements of atmospheric CO2 since 1958. The initial value was 315 ppm. The Keeling curve, named for the observatory founder, shows that value has steadily increased, exponentially, to over 417 ppm now. Compared to proxy data from atmospheric gases trapped in ice cores that show a maximum value for CO2 of about 300 ppm over the last 800,000 years, the Keeling increase of over 100 ppm in 50 years is dramatic evidence of human caused CO2 increase and climate change! As Earth’s temperature rises, glaciers and ice sheets are shrinking resulting in sea level rise. Atmospheric CO2 is also absorbed in sea water producing increased concentrations of carbonic acid which is raising the pH of the oceans making it harder for marine life to extract carbonate for their skeletal materials.
Earth’s climate has changed over geologic time with periods of major glaciations. There was a high temperature period in the Mesozoic shown by fossils in high latitudes and the Western Interior Seaway covering what is now the Midwest. However, climate has been cooling during the Cenozoic culminating in the Ice Age. Since the Ice Age, several proxy indicators of ancient climate show that the rate and amount of current climate change is unique in geologic history and can only be attributed to human activity. Those who ignore the consequences of increasing global warming for our planet’s future do so at the peril of our posterity!
Take this quiz to check your comprehension of this Chapter.
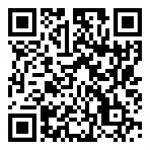
References
- Allen, P.A., and Etienne, J.L., 2008, Sedimentary challenge to Snowball Earth: Nat. Geosci., v. 1, no. 12, p. 817–825.
- Berner, R.A., 1998, The carbon cycle and carbon dioxide over Phanerozoic time: the role of land plants: Philos. Trans. R. Soc. Lond. B Biol. Sci., v. 353, no. 1365, p. 75–82.
- Cunningham, W.L., Leventer, A., Andrews, J.T., Jennings, A.E., and Licht, K.J., 1999, Late Pleistocene–Holocene marine conditions in the Ross Sea, Antarctica: evidence from the diatom record: The Holocene, v. 9, no. 2, p. 129–139.
- Deynoux, M., Miller, J.M.G., and Domack, E.W., 2004, Earth’s Glacial Record: World and Regional Geology, Cambridge University Press, World and Regional Geology.
- Earle, S., 2015, Physical geology OER textbook: BC Campus OpenEd.
- Eyles, N., and Januszczak, N., 2004, “Zipper-rift”: a tectonic model for Neoproterozoic glaciations during the breakup of Rodinia after 750 Ma: Earth-Sci. Rev.
- Francey, R.J., Allison, C.E., Etheridge, D.M., Trudinger, C.M., and others, 1999, A 1000‐year high precision record of δ13C in atmospheric CO2: Tellus B Chem. Phys. Meteorol.
- Gutro, R., 2005, NASA – What’s the Difference Between Weather and Climate? Online, http://www.nasa.gov/mission_pages/noaa-n/climate/climate_weather.html, accessed September 2016.
- Hoffman, P.F., Kaufman, A.J., Halverson, G.P., and Schrag, D.P., 1998, A neoproterozoic snowball earth: Science, v. 281, no. 5381, p. 1342–1346.
- Kopp, R.E., Kirschvink, J.L., Hilburn, I.A., and Nash, C.Z., 2005, The Paleoproterozoic snowball Earth: a climate disaster triggered by the evolution of oxygenic photosynthesis: Proc. Natl. Acad. Sci. U. S. A., v. 102, no. 32, p. 11131–11136.
- Lean, J., Beer, J., and Bradley, R., 1995, Reconstruction of solar irradiance since 1610: Implications for climate cbange: Geophys. Res. Lett., v. 22, no. 23, p. 3195–3198.
- Levitus, S., Antonov, J.I., Wang, J., Delworth, T.L., Dixon, K.W., and Broccoli, A.J., 2001, Anthropogenic warming of Earth’s climate system: Science, v. 292, no. 5515, p. 267–270.
- Lindsey, R., 2009, Climate and Earth’s Energy Budget : Feature Articles: Online, http://earthobservatory.nasa.gov, accessed September 2016.
- North Carolina State University, 2013a, Composition of the Atmosphere:
- North Carolina State University, 2013b, Composition of the Atmosphere: Online, http://climate.ncsu.edu/edu/k12/.AtmComposition, accessed September 2016.
- Oreskes, N., 2004, The scientific consensus on climate change: Science, v. 306, no. 5702, p. 1686–1686.
- Pachauri, R.K., Allen, M.R., Barros, V.R., Broome, J., Cramer, W., Christ, R., Church, J.A., Clarke, L., Dahe, Q., Dasgupta, P., Dubash, N.K., Edenhofer, O., Elgizouli, I., Field, C.B., and others, 2014, Climate Change 2014: Synthesis Report. Contribution of Working Groups I, II and III to the Fifth Assessment Report of the Intergovernmental Panel on Climate Change (R. K. Pachauri & L. Meyer, Eds.): Geneva, Switzerland, IPCC, 151 p.
- Santer, B.D., Mears, C., Wentz, F.J., Taylor, K.E., Gleckler, P.J., Wigley, T.M.L., Barnett, T.P., Boyle, J.S., Brüggemann, W., Gillett, N.P., Klein, S.A., Meehl, G.A., Nozawa, T., Pierce, D.W., and others, 2007, Identification of human-induced changes in atmospheric moisture content: Proc. Natl. Acad. Sci. U. S. A., v. 104, no. 39, p. 15248–15253.
- Schopf, J.W., and Klein, C., 1992, Late Proterozoic Low-Latitude Global Glaciation: the Snowball Earth, in Schopf, J.W., and Klein, C., editors, The Proterozoic biosphere : a multidisciplinary study: New York, Cambridge University Press, p. 51–52.
- Webb, T., and Thompson, W., 1986, Is vegetation in equilibrium with climate? How to interpret late-Quaternary pollen data: Vegetatio, v. 67, no. 2, p. 75–91.
- Weissert, H., 2000, Deciphering methane’s fingerprint: Nature, v. 406, no. 6794, p. 356–357.
- Whitlock, C., and Bartlein, P.J., 1997, Vegetation and climate change in northwest America during the past 125 kyr: Nature, v. 388, no. 6637, p. 57–61.
- Wolpert, S., 2009, New NASA temperature maps provide a ‘whole new way of seeing the moon’: Online, http://newsroom.ucla.edu/releases/new-nasa-temperature-maps-provide-102070, accessed February 2017.
- Zachos, J., Pagani, M., Sloan, L., Thomas, E., and Billups, K., 2001, Trends, rhythms, and aberrations in global climate 65 Ma to present: Science, v. 292, no. 5517, p. 686–693.
Mention de la source du contenu multimédia
- The_Earth_seen_from_Apollo_17
- Atmosphere_gas_proportions
- Carbon Cycle
- Solar_Spectrum
- reflected_radiation
- surface_energy_balance
- Net-Forcings
- 15.1 Did I Get It QR Code
- Evidence for Climate Change Youtube QR Code
- tempgraph
- 15.2.1 Global Temperature Rise Video QR Code
- Keeling-Curve-1-10-2022-1
- Pumphandle 2016 Youtube QR Code
- LandIceAntarctica
- Watch 25 Years of Arctic Sea Ice Disappear Youtube QR Code
- Ball and Ring Rate of Expansion and Contraction Youtube QR Code
- 15.2.5 Ocean Acidification Video QR Code
- 15.2 Did I Get It QR Code
- Laurentide_Ice_Sheet_Extent
- cenozoic-t-2
- Antarctic-Circumpolar
- oxygen isotope record Lisiecki and Raymo 2005
- sediment-core_hg
- Ice_Age_Temperature
- GISP2_1855m_ice_core_layers
- Air_Bubbles_Trapped_in_Ice
- Co2_glacial_cycles_800k
- Climatic Evidence From Sediments Youtube QR Code
- Tree.rings_
- Tree Ring Temperature Anamoly_Yamal50
- Misc_pollen_colorized
- 15.3 Did I Get It QR Code
- Fig 1.7 from Pachauri et al 2014
- 15.4_Fig 1.5 Pachauri et al. 2014 emissions 1750-2011
- 15.4 Did I Get It QR Code
- Ch.15 Review QR Code
Photo credit to Louis J. Maher, Jr.
Sedimentary rocks made of mineral grains weathered as mechanical detritus of previous rocks, e.g. sand, gravel, etc.
Martyn Gorman [CC BY-SA 2.0], via Wikimedia Commons
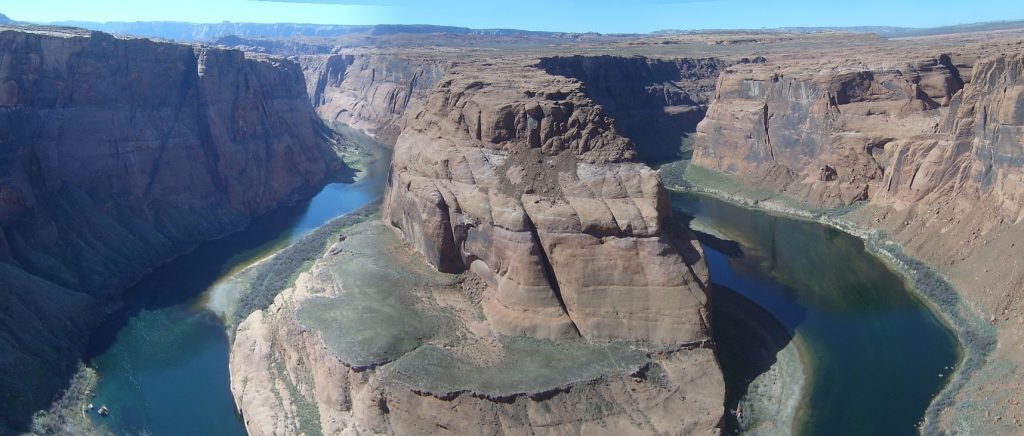
11 Water
KEY CONCEPTS
-
Example of a Roman aqueduct in Segovia, Spain. Describe the processes of the water cycle
- Describe drainage basins, watershed protection, and water budget
- Describe reasons for water laws, who controls them, and how water is shared in the western U.S.
- Describe zone of transport, zone of sediment production, zone of deposition, and equilibrium
- Describe stream landforms: channel types, alluvial fans, floodplains, natural levees, deltas, entrenched meanders, and terraces
- Describe the properties required for a good aquifer; define confining layer water table
- Describe three major groups of water contamination and three types of remediation
- Describe karst topography, how it is created, and the landforms that characterize it
All life on Earth requires water. The hydrosphere (Earth’s water) is an important agent of geologic change. Water shapes our planet by depositing minerals, aiding lithification, and altering rocks after they are lithified. Water carried by subducted oceanic plates causes flux melting of upper mantle material. Water is among the volatiles in magma and emerges at the surface as steam in volcanoes.
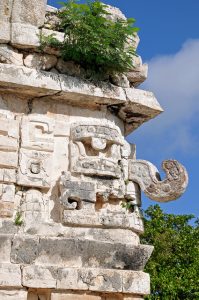
Humans rely on suitable water sources for consumption, agriculture, power generation, and many other purposes. In pre-industrial civilizations, the powerful controlled water resources [1, 2]. As shown in the figures, two thousand year old Roman aqueducts still grace European, Middle Eastern, and North African skylines. Ancient Mayan architecture depicts water imagery such as frogs, water-lilies, water fowl to illustrate the importance of water in their societies [3]. In the drier lowlands of the Yucatan Peninsula, mask facades of the hooked-nosed rain god, Chac (or Chaac) are prominent on Mayan buildings such as the Kodz Poop (Temple of the Masks, sometimes spelled Coodz Poop) at the ceremonial site of Kabah. To this day government controlled water continues to be an integral part of most modern societies.
11.1 Water Cycle
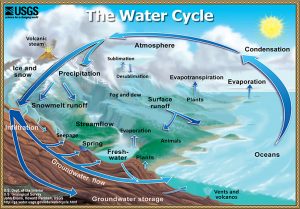
The water cycle is the continuous circulation of water in the Earth's atmosphere. During circulation, water changes between solid, liquid, and gas (water vapor) and changes location. The processes involved in the water cycle are evaporation, transpiration, condensation, precipitation, and runoff.
Evaporation is the process by which a liquid is converted to a gas. Water evaporates when solar energy warms the water sufficiently to excite the water molecules to the point of vaporization. Evaporation occurs from oceans, lakes, and streams and the land surface. Plants contribute significant amounts of water vapor as a byproduct of photosynthesis called transpiration that occurs through the minute pores of plant leaves. The term evapotranspiration refers to these two sources of water entering the atmosphere and is commonly used by geologists.
Water vapor is invisible. Condensation is the process of water vapor transitioning to a liquid. Winds carry water vapor in the atmosphere long distances. When water vapor cools or when air masses of different temperatures mix, water vapor may condense back into droplets of liquid water. These water droplets usually form around a microscopic piece of dust or salt called condensation nuclei. These small droplets of liquid water suspended in the atmosphere become visible as in a cloud. Water droplets inside clouds collide and stick together, growing into larger droplets. Once the water droplets become big enough, they fall to Earth as rain, snow, hail, or sleet.
Once precipitation has reached the Earth's surface, it can evaporate or flow as runoff into streams, lakes, and eventually back to the oceans. Water in streams and lakes is called surface water. Or water can also infiltrate into the soil and fill the pore spaces in the rock or sediment underground to become groundwater. Groundwater slowly moves through rock and unconsolidated materials. Some groundwater may reach the surface again, where it discharges as springs, streams, lakes, and the ocean. Also, surface water in streams and lakes can infiltrate again to recharge groundwater. Therefore, the surface water and groundwater systems are connected.
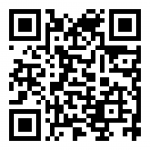
Take this quiz to check your comprehension of this section.
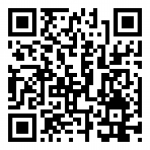
11.2 Water Basins and Budgets
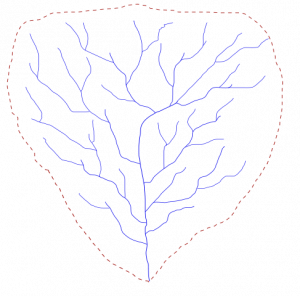
The basic unit of division of the landscape is the drainage basin, also known as a catchment or watershed. It is the area of land that captures precipitation and contributes runoff to a stream or stream segment [4]. Drainage divides are local topographic high points that separate one drainage basin from another [5]. Water that falls on one side of the divide goes to one stream, and water that falls on the other side of the divide goes to a different stream. Each stream, tributary and streamlet has its own drainage basin. In areas with flatter topography, drainage divides are not as easily identified but they still exist [6].
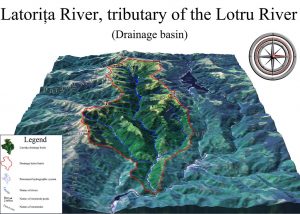
The headwater is where the stream begins. Smaller tributary streams combine downhill to make the larger trunk of the stream. The mouth is where the stream finally reaches its end. The mouth of most streams is at the ocean. However, a rare number of streams do not flow to the ocean, but rather end in a closed basin (or endorheic basin) where the only outlet is evaporation. Most streams in the Great Basin of Western North America end in endorheic basins. For example, in Salt Lake County, Utah, Little Cottonwood Creek and the Jordan River flow into the endorheic Great Salt Lake where the water evaporates.
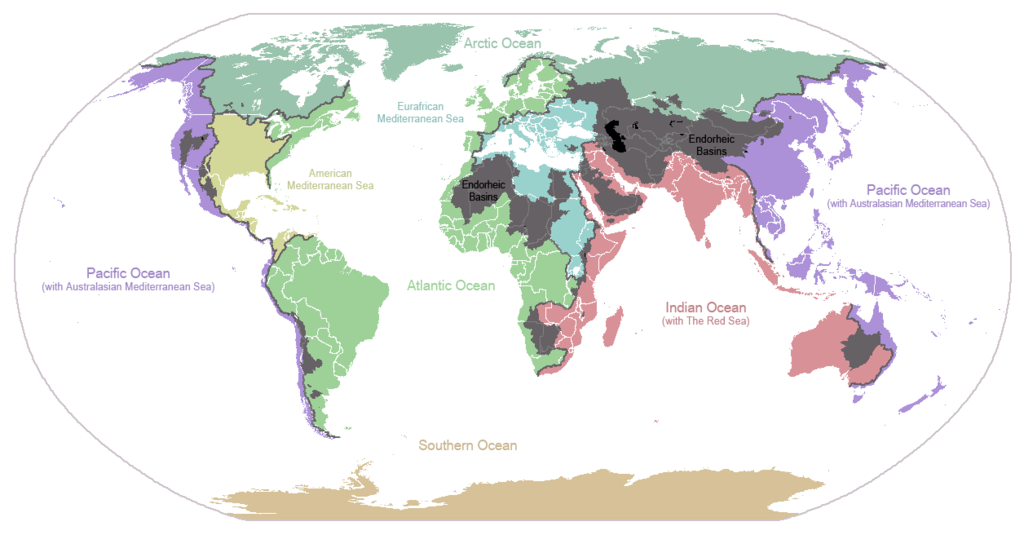
Perennial streams flow all year round. Perennial streams occur in humid or temperate climates where there is sufficient rainfall and low evaporation rates. Water levels rise and fall with the seasons, depending on the discharge. Ephemeral streams flow only during rain events or the wet season. In arid climates, like Utah, many streams are ephemeral. These streams occur in dry climates with low amounts of rainfall and high evaporation rates. Their channels are often dry washes or arroyos for much of the year and their sudden flow causes flash floods [7].
Along Utah’s Wasatch Front, the urban area extending north to south from Brigham City to Provo, there are several watersheds that are designated as “watershed protection areas” that limit the type of use allowed in those drainages in order to protect culinary water. Dogs and swimming are limited in those watersheds because of the possibility of contamination by harmful bacteria and substances to the drinking supply of Salt Lake City and surrounding municipalities.
Water in the water cycle is very much like money in a personal budget. Income includes precipitation and stream and groundwater inflow. Expenses include groundwater withdrawal, evaporation, and stream and groundwater outflow. If the expenses outweigh the income, the water budget is not balanced. In this case, water is removed from savings, i.e. water storage, if available. Reservoirs, snow, ice, soil moisture, and aquifers all serve as storage in a water budget. In dry regions, the water is critical for sustaining human activities. Understanding and managing the water budget is an ongoing political and social challenge.
Hydrologists create groundwater budgets within any designated area, but they are generally made for watershed (basin) boundaries, because groundwater and surface water are easier to account for within these boundaries. Water budgets can be created for state, county, or aquifer extent boundaries as well. The groundwater budget is an essential component of the hydrologic model; hydrologists use measured data with a conceptual workflow of the model to better understand the water system.
Take this quiz to check your comprehension of this section.
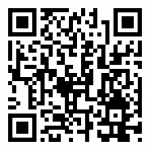
11.3 Water Use and Distribution
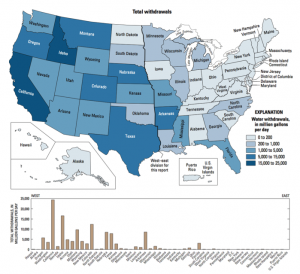
In the United States, 1,344 billion L (355 billion gallons) of ground and surface water are used each day, of which 288 billion L (76 billion gallons) are fresh groundwater. The state of California uses 16% of national groundwater [8].
Utah is the second driest state in the United States. Nevada, having a mean statewide precipitation of 31 cm (12.2 inches) per year, is the driest. Utah also has the second highest per capita rate of total domestic water use of 632.16 L (167 gallonsL per day per person [8]. With the combination of relatively high demand and limited quantity, Utah is at risk for water budget deficits.
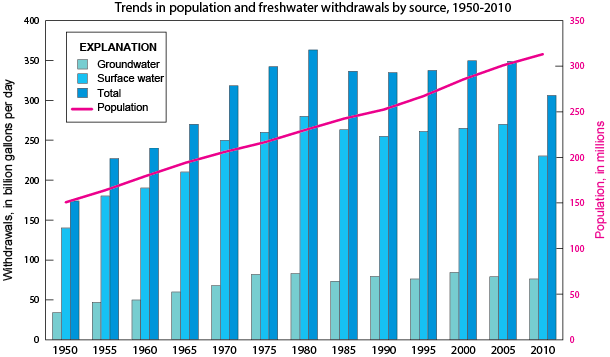
11.3.1 Surface Water Distribution
Fresh water is a precious resource and should not be taken for granted, especially in dry climates. Surface water makes up only 1.2% of the fresh water available on the planet, and 69% of that surface water is trapped in ground ice and permafrost. Stream water accounts for only 0.006% of all freshwater and lakes contain only 0.26% of the world’s fresh water [9].
Global circulation patterns are the most important factor in distributing surface water through precipitation. Due to the Coriolis effect and the uneven heating of the Earth, air rises near the equator and near latitudes 60° north and south. Air sinks at the poles and latitudes 30° north and south (see Chapter 13). Land masses near rising air are more prone to humid and wet climates. Land masses near sinking air, which inhibits precipitation, are prone to dry conditions [10, 11]. Prevailing winds, ocean circulation patterns such as the Gulf Stream’s effects on eastern North America, rain shadows (the dry leeward sides of mountains), and even the proximity of bodies of water can affect local climate patterns. When this moist air collides with the nearby mountains causing it to rise and cool, the moisture may fall out as snow or rain on nearby areas in a phenomenon known as “lake-effect precipitation.” [12]
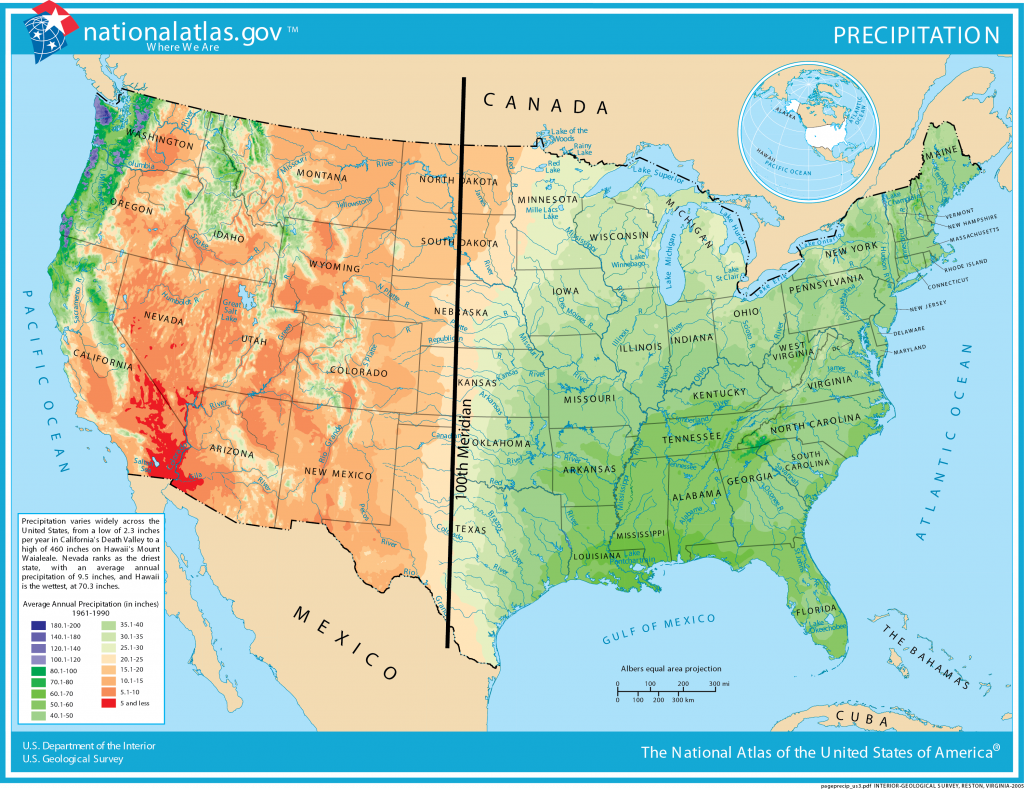
In the United States, the 100th meridian roughly marks the boundary between the humid and arid parts of the country. Growing crops west of the 100th meridian requires irrigation [13]. In the west, surface water is stored in reservoirs and mountain snowpacks [14], then strategically released through a system of canals during times of high water use.
Some of the driest parts of the western United States are in the Basin and Range Province. The Basin and Range has multiple mountain ranges that are oriented north to south. Most of the basin valleys in the Basin and Range are dry, receiving less than 30 cm (12 inches) of precipitation per year. However, some of the mountain ranges can receive more than 1.52 m (60 inches) of water as snow or snow-water-equivalent. The snow-water equivalent is the amount of water that would result if the snow were melted, as the snowpack is generally much thicker than the equivalent amount of water that it would produce [12].
11.3.2 Groundwater Distribution
Water source | Water volume
(cubic miles) |
Fresh water (%) | Total water (%) |
---|---|---|---|
Oceans, Seas, & Bays | 321,000,000 | -- | 96.5 |
Ice caps, Glaciers, & Permanent Snow | 5,773,000 | 68.7 | 1.74 |
Groundwater | 5,614,000 | -- | 1.69 |
-- Fresh | 2,526,000 | 30.1 | 0.76 |
-- Saline | 3,088,000 | -- | 0.93 |
Soil Moisture | 3,959 | 0.05 | 0.001 |
Ground Ice & Permafrost | 71,970 | 0.86 | 0.022 |
Lakes | 42,320 | -- | 0.013 |
-- Fresh | 21,830 | 0.26 | 0.007 |
-- Saline | 20,490 | -- | 0.006 |
Atmosphere | 3,095 | 0.04 | 0.001 |
Swamp Water | 2,752 | 0.03 | 0.0008 |
Rivers | 509 | 0.006 | 0.0002 |
Biological Water | 269 | 0.003 | 0.0001 |
Source: Igor Shiklomanov's chapter "World fresh water resources" in Peter H. Gleick (editor), 1993, Water in Crisis: A Guide to the World's Fresh Water Resources (Oxford University Press, New York)[zotpressInText item="{P7VGIQT4}" format="%num%" brackets="yes" separator="comma"] |
Groundwater makes up 30.1% of the fresh water on the planet, making it the most abundant reservoir of fresh water accessible to most humans. The majority of freshwater, 68.7%, is stored in glaciers and ice caps as ice [9]. As the glaciers and ice caps melt due to global warming, this fresh water is lost as it flows into the oceans.
Take this quiz to check your comprehension of this section.
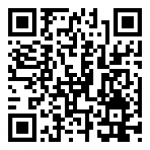
11.4 Water Law
Federal and state governments have put laws in place to ensure the fair and equitable use of water. In the United States, the states are tasked with creating a fair and legal system for sharing water.
11.4.1 Water Rights
Because of the limited supply of water, especially in the western United States, states disperse a system of legal water rights defined as a claim to a portion or all of a water source, such as a spring, stream, well, or lake. Federal law mandates that states control water rights, with the special exception of federally reserved water rights, such as those associated with national parks and Native American tribes, and navigation servitude that maintains navigable water bodies. Each state in the United States has a different way to disperse and manage water rights.
A person, entity, company, or organization, must have a water right to legally extract or use surface or groundwater in their state. Water rights in some western states are dictated by the concept of prior appropriation, or “first in time, first in right,” where the person with the oldest water right gets priority water use during times when there is not enough water to fulfill every water right.
The Colorado River and its tributaries pass through a desert region, including seven states (Wyoming, Colorado, Utah, New Mexico, Arizona, Nevada, California), Native American reservations, and Mexico. As the western United States became more populated and while California was becoming a key agricultural producer, the states along the Colorado River realized that the river was important to sustaining life in the West.
To guarantee certain perceived water rights, these western states recognized that a water budget was necesary for the Colorado River Basin. Thus was enacted the Colorado River Compact in 1922 to ensure that each state got a fair share of the river water. The Compact granted each state a specific volume of water based on the total measured flow at the time. However, in 1922, the flow of the river was higher than its long-term average flow, consequently, more water was allocated to each state than is typically available in the river [16].
Over the next several decades, lawmakers have made many other agreements and modifications regarding the Colorado River Compact, including those agreements that brought about the Hoover Dam (formerly Boulder Dam), and Glen Canyon Dam, and a treaty between the American and Mexican governments. Collectively, the agreements are referred to as “The Law of the River" by the United States Bureau of Reclamation. Despite adjustments to the Colorado River Compact, many believe that the Colorado River is still over-allocated, as the Colorado River flow no longer reaches the Pacific Ocean, its original terminus (base level). Dams along the Colorado River have caused water to divert and evaporate, creating serious water budget concerns in the Colorado River Basin. Predicted drought associated with global warming is causing additional concerns about over-allocating the Colorado River flow in the future.
The Law of the River highlights the complex and prolonged nature of interstate water rights agreements, as well as the importance of water.
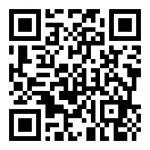
The Snake Valley straddles the border of Utah and Nevada with more of the irrigable land area lying on the Utah side of the border. In 1989, the Southern Nevada Water Authority (SNWA) submitted applications for water rights to pipe up to 191,189,707 cu m (155,000 ac-ft) of water per year (an acre-foot of water is one acre covered with water one foot deep) from Spring, Snake, Delamar, Dry Lake, and Cave valleys to southern Nevada, mostly for Las Vegas [17]. Nevada and Utah have attempted a comprehensive agreement, but negotiations have not yet been settled.
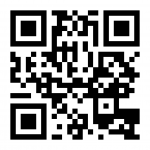
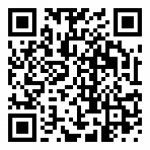
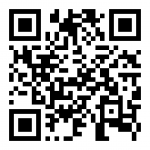
11.4.2 Water Quality and Protection
Two major federal laws that protect water quality in the United States are the Clean Water Act and the Safe Drinking Water Act. The Clean Water Act, an amendment of the Federal Water Pollution Control Act, protects navigable waters from dumping and point-source pollution. The Safe Drinking Water Act ensures that water that is provided by public water suppliers, like cities and towns, is safe to drink [18].
The U.S. Environmental Protection Agency Superfund program ensures the cleanup of hazardous contamination, and can be applied to situations of surface water and groundwater contamination. It is part of the Comprehensive Environmental Response, Compensation, and Liability Act of 1980. Under this act, state governments and the U.S. Environmental Protection Agency can use the superfund to pay for remediation of a contaminated site and then file a lawsuit against the polluter to recoup the costs. Or to avoid being sued, the polluter that caused the contamination may take direct action or provide funds to remediate the contamination.
Take this quiz to check your comprehension of this section.
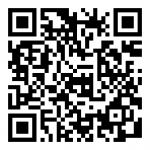
11.5 Surface Water
Geologically, a stream is a body of flowing surface water confined to a channel. Terms such as river, creek and brook are social terms not used in geology. Streams erode and transport sediments, making them the most important agents of the earth’s surface, along with wave action (see Chapter 12) in eroding and transporting sediments. They create much of the surface topography and are an important water resource.
Several factors cause streams to erode and transport sediment, but the two main factors are stream-channel gradient and velocity. Stream-channel gradient is the slope of the stream usually expressed in meters per kilometer or feet per mile. A steeper channel gradient promotes erosion. When tectonic forces elevate a mountain, the stream gradient increases, causing the mountain stream to erode downward and deepen its channel eventually forming a valley. Stream-channel velocity is the speed at which channel water flows. Factors affecting channel velocity include channel gradient which decreases downstream, discharge and channel size which increase as tributaries coalesce, and channel roughness which decreases as sediment lining the channel walls decreases in size thus reducing friction. The combined effect of these factors is that channel velocity actually increases from mountain brooks to the mouth of the stream.
11.5.1 Discharge
Stream size is measured in terms of discharge, the volume of water flowing past a point in the stream over a defined time interval. Volume is commonly measured in cubic units (length x width x depth), shown as feet3 (ft3) or meter3 (m3). Therefore, the units of discharge are cubic feet per second (ft3/sec or cfs). Therefore, the units of discharge are cubic meters per second, (m³/s or cms, or cubic feet per second (ft³/sec or cfs). Stream discharge increases downstream. Smaller streams have less discharge than larger streams. For example, the Mississippi River is the largest river in North America, with an average flow of about 16,990.11 cms (600,000 cfs) [19]. For comparison, the average discharge of the Jordan River at Utah Lake is about 16.25 cms (574 cfs) [20] and for the annual discharge of the Amazon River, (the world’s largest river), annual discharge is about 175,565 cms (6,200,000 cfs) [21].
Discharge can be expressed by the following equation:
Q = V A
- Q = discharge cms (or ft3/sec),
- A = cross-sectional area of the stream channel [width times average depth] as m2 (or in2 or ft2),
- V = average channel velocity m/s (or ft/sec) [7]
At a given location along the stream, velocity varies with stream width, shape, and depth within the stream channel as well. When the stream channel narrows but discharge remains constant, the same volume of water must flows through a narrower space causing the velocity to increase, similar to putting a thumb over the end of a backyard water hose. In addition, during rain storms or heavy snow melt, runoff increases, which increases stream discharge and velocity.
When the stream channel curves, the highest velocity will be on the outside of the bend. When the stream channel is straight and uniformly deep, the highest velocity is in the channel center at the top of the water where it is the farthest from frictional contact with the stream channel bottom and sides. In hydrology, the thalweg of a river is the line drawn that shows its natural progression and deepest channel, as is shown in the diagram.
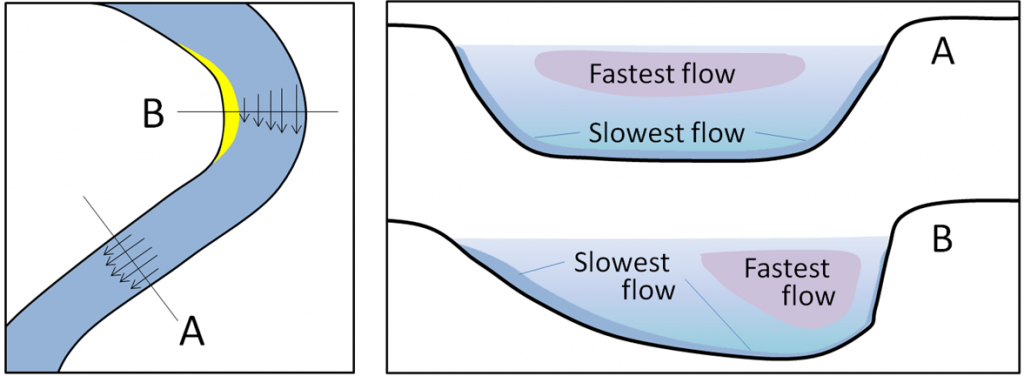
11.5.2 Runoff vs. Infiltration
Factors that dictate whether water will infiltrate into the ground or run off over the land include the amount, type, and intensity of precipitation; the type and amount of vegetation cover; the slope of the land; the temperature and aspect of the land; preexisting conditions; and the type of soil in the infiltrated area. High- intensity rain will cause more runoff than the same amount of rain spread out over a longer duration. If the rain falls faster than the soil’s properties allow it to infiltrate, then the water that cannot infiltrate becomes runoff. Dense vegetation can increase infiltration, as the vegetative cover slows the water particle’s overland flow giving them more time to infiltrate. If a parcel of land has more direct solar radiation or higher seasonal temperatures, there will be less infiltration and runoff, as evapotranspiration rates will be higher. As the land’s slope increases, so does runoff, because the water is more inclined to move downslope than infiltrate into the ground. Extreme examples are a basin and a cliff, where water infiltrates much quicker into a basin than a cliff that has the same soil properties. Because saturated soil does not have the capacity to take more water, runoff is generally greater over saturated soil. Clay-rich soil cannot accept infiltration as quickly as gravel-rich soil.
11.5.3 Drainage Patterns
The pattern of tributaries within a region is called drainage pattern. They depend largely on the type of rock beneath, and on structures within that rock (such as folds and faults). The main types of drainage patterns are dendritic, trellis, rectangular, radial, and deranged. Dendritic patterns are the most common and develop in areas where the underlying rock or sediments are uniform in character, mostly flat lying, and can be eroded equally easily in all directions. Examples are alluvial sediments or flat lying sedimentary rocks. Trellis patterns typically develop where sedimentary rocks have been folded or tilted and then eroded to varying degrees depending on their strength. The Appalachian Mountains in eastern United States have many good examples of trellis drainage. Rectangular patterns develop in areas that have very little topography and a system of bedding planes, joints, or faults that form a rectangular network. A radial pattern forms when streams flow away from a central high point such as a mountain top or volcano, with the individual streams typically having dendritic drainage patterns. In places with extensive limestone deposits, streams can disappear into the groundwater via caves and subterranean drainage and this creates a deranged pattern [4].

11.5.4 Fluvial Processes
Fluvial processes dictate how a stream behaves and include factors controlling fluvial sediment production, transport, and deposition. Fluvial processes include velocity, slope and gradient, erosion, transportation, deposition, stream equilibrium, and base level.
Streams can be divided into three main zones: the many smaller tributaries in the source area, the main trunk stream in the floodplain and the distributaries at the mouth of the stream. Major stream systems like the Mississippi are composed of many source areas, many tributaries and trunk streams, all coalescing into the one main stream draining the region. The zones of a stream are defined as 1) the zone of sediment production (erosion), 2) the zone of transport, and 3) the zone of deposition. The zone of sediment production is located in the headwaters of the stream. In the zone of sediment transport, there is a general balance between erosion of the finer sediment in its channel and transport of sediment across the floodplain. Streams eventually flow into the ocean or end in quiet water with a delta which is a zone of sediment deposition located at the mouth of a stream [6]. The longitudinal profile of a stream is a plot of the elevation of the stream channel at all points along its course and illustrates the location of the three zones [22]
Zone of Sediment Production
The zone of sediment production is located in the headwaters of a stream where rills and gullies erode sediment and contribute to larger tributary streams. These tributaries carry sediment and water further downstream to the main trunk of the stream. Tributaries at the headwaters have the steepest gradient; erosion there produces considerable sediment carried b the stream. Headwater streams tend to be narrow and straight with small or non-existent floodplains adjacent to the channel. Since the zone of sediment production is generally the steepest part of the stream, headwaters are generally located in relatively high elevations. The Rocky Mountains of Wyoming and Colorado west of the Continental Divide contain much of the headwaters for the Colorado River which then flows from Colorado through Utah and Arizona to Mexico. Headwaters of the Mississippi river system lie east of the Continental Divide in the Rocky Mountains and west of the Appalachian Divide.
Zone of Sediment TransPORT
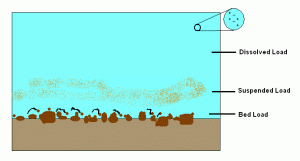
Streams transport sediment great distances from the headwaters to the ocean, the ultimate depositional basins. Sediment transportation is directly related to stream gradient and velocity. Faster and steeper streams can transport larger sediment grains. When velocity slows down, larger sediments settle to the channel bottom. When the velocity increases, those larger sediments are entrained and move again.
Transported sediments are grouped into bedload, suspended load, and dissolved load as illustrated in the above image. Sediments moved along the channel bottom are the bedload that typically consists of the largest and densest particles. Bedload is moved by saltation (bouncing) and traction (being pushed or rolled along by the force of the flow). Smaller particles are picked up by flowing water and carried in suspension as suspended load. The particle size that is carried in suspended and bedload depends on the flow velocity of the stream. Dissolved load in a stream is the total of the ions in solution from chemical weathering, including such common ions such as bicarbonate (-HCO3-), calcium (Ca+2), chloride (Cl-1), potassium (K+1), and sodium (Na+1). The amounts of these ions are not affected by flow velocity.
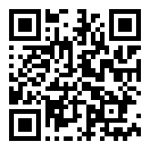

A floodplain is the flat area of land adjacent to a stream channel inundated with flood water on a regular basis. Stream flooding is a natural process that adds sediment to floodplains. A stream typically reaches its greatest velocity when it is close to flooding, known as the bankfull stage. As soon as the flooding stream overtops its banks and flows onto its floodplain, the velocity decreases. Sediment that was being carried by the swiftly moving water is deposited at the edge of the channel, forming a low ridge or natural levée. In addition, sediments are added to the floodplain during this flooding process contributing to fertile soils [4].
Zone of SEDIMENT Deposition
Deposition occurs when bedload and suspended load come to rest on the bottom of the stream channel, lake, or ocean due to decrease in stream gradient and reduction in velocity. While both deposition and erosion occur in the zone of transport such as on point bars and cut banks, ultimate deposition where the stream reaches a lake or ocean. Landforms called deltas form where the stream enters quiet water composed of the finest sediment such as fine sand, silt, and clay.
Equilibrium and Base Level
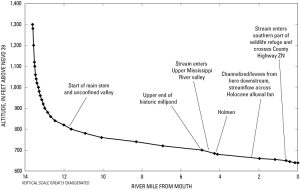
All three stream zones are present in the typical longitudinal profile of a stream which plots the elevation of the channel at all points along its course (see figure). All streams have a long profile. The long profile shows the stream gradient from headwater to mouth. All streams attempt to achieve an energetic balance among erosion, transport, gradient, velocity, discharge, and channel characteristics along the stream’s profile. This balance is called equilibrium, a state called grade.
Another factor influencing equilibrium is base level, the elevation of the stream's mouth representing the lowest level to which a stream can erode. The ultimate base level is, of course, sea-level. A lake or reservoir may also represent base level for a stream entering it. The Great Basin of western Utah, Nevada, and parts of some surrounding states contains no outlets to the sea and provides internal base levels for streams within it. Base level for a stream entering the ocean changes if sea-level rises or falls. Base level also changes if a natural or human-made dam is added along a stream's profile. When base level is lowered, a stream will cut down and deepen its channel. When base level rises, deposition increases as the stream adjusts attempting to establish a new state of equilibrium. A stream that has approximately achieved equilibrium is called a graded stream.
11.5.5 Fluvial Landforms
Stream landforms are the land features formed on the surface by either erosion or deposition. The stream-related landforms described here are primarily related to channel types.
Channel Types
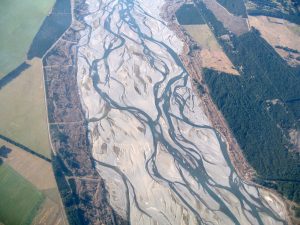
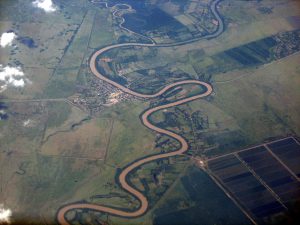
Stream channels can be straight, braided, meandering, or entrenched. The gradient, sediment load, discharge, and location of base level all influence channel type. Straight channels are relatively straight, located near the headwaters, have steep gradients, low discharge, and narrow V-shaped valleys. Examples of these are located in mountainous areas.
Braided streams have multiple channels splitting and recombining around numerous mid-channel bars. These are found in floodplains with low gradients in areas with near sources of coarse sediment such as trunk streams draining mountains or in front of glaciers.
Meandering streams have a single channel that curves back and forth like a snake within its floodplain where it emerges from its headwaters into the zone of transport. Meandering streams are dynamic creating a wide floodplain by eroding and extending meander loops side-to-side. The highest velocity water is located on the outside of a meander bend. Erosion of the outside of the curve creates a feature called a cut bank and the meander extends its loop wider by this erosion.
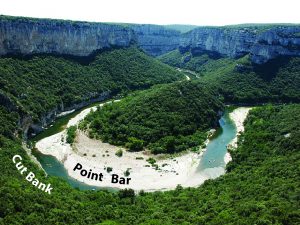
The thalweg of the stream is the deepest part of the stream channel. In the straight parts of the channel, the thalweg and highest velocity are in the center of the channel. But at the bend of a meandering stream, the thalweg shifts toward the cut bank. Opposite the cutbank on the inside bend of the channel is the lowest stream velocity and is an area of deposition called a point bar.
In areas of tectonic uplift such as on the Colorado Plateau, meandering streams that once flowed on the plateau surface have become entrenched or incised as uplift occurred and the stream cut its meandering channel down into bedrock. Over the past several million years, the Colorado River and its tributaries have incised into the flat lying rocks of the plateau by hundreds, even thousands of feet creating deep canyons including the Grand Canyon in Arizona.
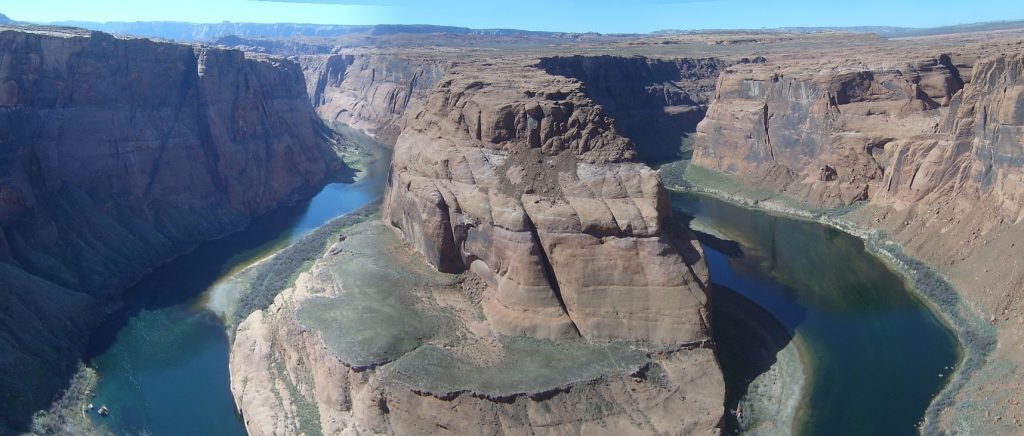
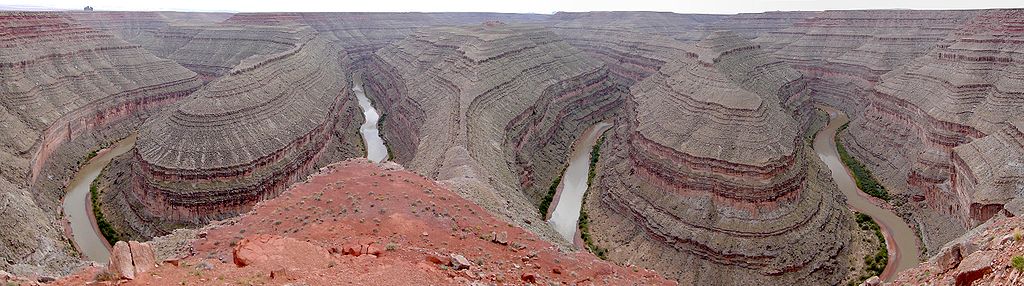
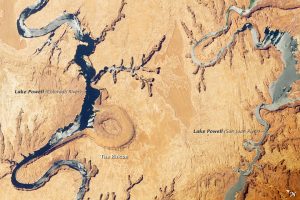
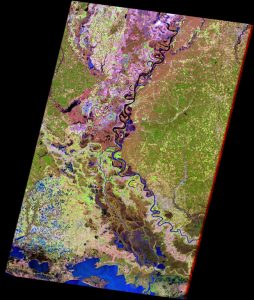
Many fluvial landforms occur on a floodplain associated with a meandering stream. Meander activity and regular flooding contribute to widening the floodplain by eroding adjacent uplands. The stream channels are confined by natural levees that have been built up over many years of regular flooding. Natural levees can isolate and direct flow from tributary channels on the floodplain from immediately reaching the main channel. These isolated streams are called yazoo streams and flow parallel to the main trunk stream until there is an opening in the levee to allow for a belated confluence.
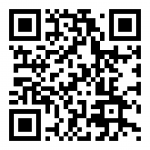
To limit flooding, humans build artificial levees on flood plains. Sediment that breaches the levees during flood stage is called crevasse splays and delivers silt and clay onto the floodplain. These deposits are rich in nutrients and often make good farm land. When floodwaters crest over human-made levees, the levees quickly erode with potentially catastrophic impacts. Because of the good soils, farmers regularly return after floods and rebuild year after year.
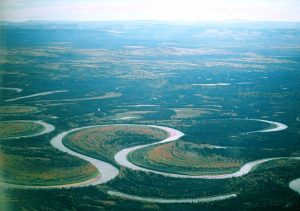
Through erosion on the outsides of the meanders and deposition on the insides, the channels of meandering streams move back and forth across their floodplain over time. On very broad floodplains with very low gradients, the meander bends can become so extreme that they cut across themselves at a narrow neck (see figure) called a cutoff. The former channel becomes isolated and forms an oxbow lake seen on the right of the figure. Eventually the oxbow lake fills in with sediment and becomes a wetland and eventually a meander scar. Stream meanders can migrate and form oxbow lakes in a relatively short amount of time. Where stream channels form geographic and political boundaries, this shifting of channels can cause conflicts.
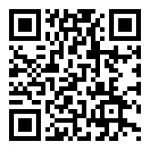
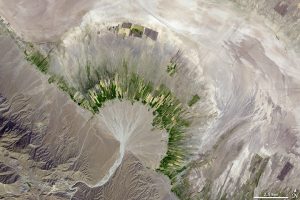
Alluvial fans are a depositional landform created where streams emerge from mountain canyons into a valley. The channel that had been confined by the canyon walls is no longer confined, slows down and spreads out, dropping its bedload of all sizes, forming a delta in the air of the valley. As distributary channels fill with sediment, the stream is diverted laterally, and the alluvial fan develops into a cone shaped landform with distributaries radiating from the canyon mouth. Alluvial fans are common in the dry climates of the West where ephemeral streams emerge from canyons in the ranges of the Basin and Range.
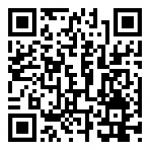
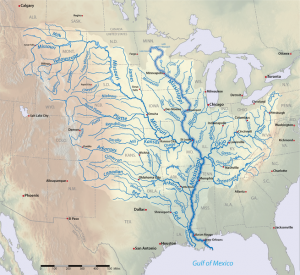
A delta is formedwhen a stream reaches a quieter body of water such as a lake or the ocean and the bedload and suspended load is deposited. If wave erosion from the water body is greater than deposition from the river, a delta will not form. The largest and most famous delta in the United States is the Mississippi River delta formed where the Mississippi River flows into the Gulf of Mexico. The Mississippi River drainage basin is the largest in North America, draining 41% of the contiguous United States [24]. Because of the large drainage area, the river carries a large amount of sediment. The Mississippi River is a major shipping route and human engineering has ensured that the channel has been artificially straightened and remains fixed within the floodplain. The river is now 229 km shorter than it was before humans began engineering it [24]. Because of these restraints, the delta is now focused on one trunk channel and has created a “bird’s foot” pattern. The two NASA images below of the delta show how the shoreline has retreated and land was inundated with water while deposition of sediment was focused at end of the distributaries. These images have changed over a 25 year period from 1976 to 2001. These are stark changes illustrating sea-level rise and land subsidence from the compaction of peat due to the lack of sediment resupply [25].
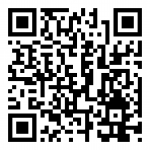
The formation of the Mississippi River delta started about 7500 years ago when postglacial sea level stopped rising. In the past 7000 years, prior to anthropogenic modifications, the Mississippi River delta formed several sequential lobes. The river abandoned each lobe for a more preferred route to the Gulf of Mexico. These delta lobes were reworked by the ocean waves of the Gulf of Mexico [26]. After each lobe was abandoned by the river, isostatic depression and compaction of the sediments caused basin subsidence and the land to sink.
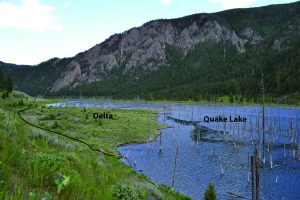
A clear example of how deltas form came from an earthquake. During the 1959 Madison Canyon 7.5 magnitude earthquake in Montana, a large landslide dammed the Madison River forming Quake Lake still there today [27]. A small tributary stream that once flowed into the Madison River, now flows into Quake Lake forming a delta composed of coarse sediment actively eroded from the mountainous upthrown block to the north.
Deltas can be further categorized as wave-dominated or tide-dominated. Wave-dominated deltas occur where the tides are small and wave energy dominates. An example is the Nile River delta in the Mediterranean Sea that has the classic shape of the Greek character (Δ) from which the landform is named. A tide-dominated delta forms when ocean tides are powerful and influence the shape of the delta. For example, Ganges-Brahmaputra Delta in the Bay of Bengal (near India and Bangladesh) is the world’s largest delta and mangrove swamp called the Sundarban [29].
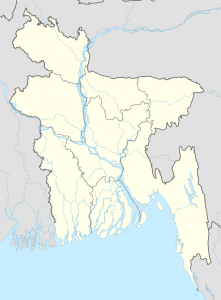
At the Sundarban Delta in Bangladesh, tidal forces create linear intrusions of seawater into the delta. This delta also holds the world’s largest mangrove swamp.
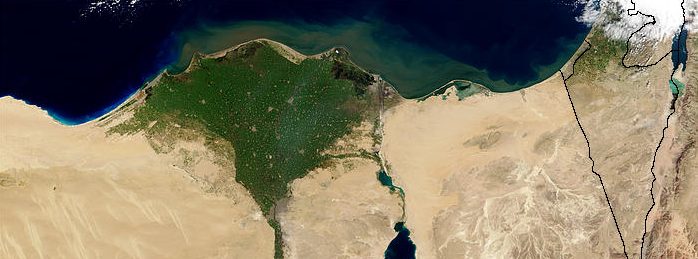
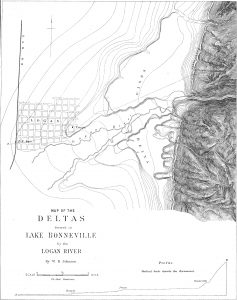
Lake Bonneville was a large, pluvial lake that occupied the western half of Utah and parts of eastern Nevada from about 30,000 to 12,000 years ago. The lake filled to a maximum elevation as great as approximately 5100 feet above mean sea level, filling the basins, leaving the mountains exposed, many as islands. The presence of the lake allowed for deposition of both fine grained lake mud and silt and coarse gravels from the mountains. Variations in lake level were controlled by regional climate and a catastrophic failure of Lake Bonneville’s main outlet, Red Rock Pass [31]. during extended periods of time in which the lake level remained stable, wave-cut terraces were produced that can be seen today on the flanks of many mountains in the region. Significant deltas formed at the mouths of major canyons in Salt Lake, Cache, and other Utah valleys. The Great Salt Lake is the remnant of Lake Bonneville and cities have built up on these delta deposits.
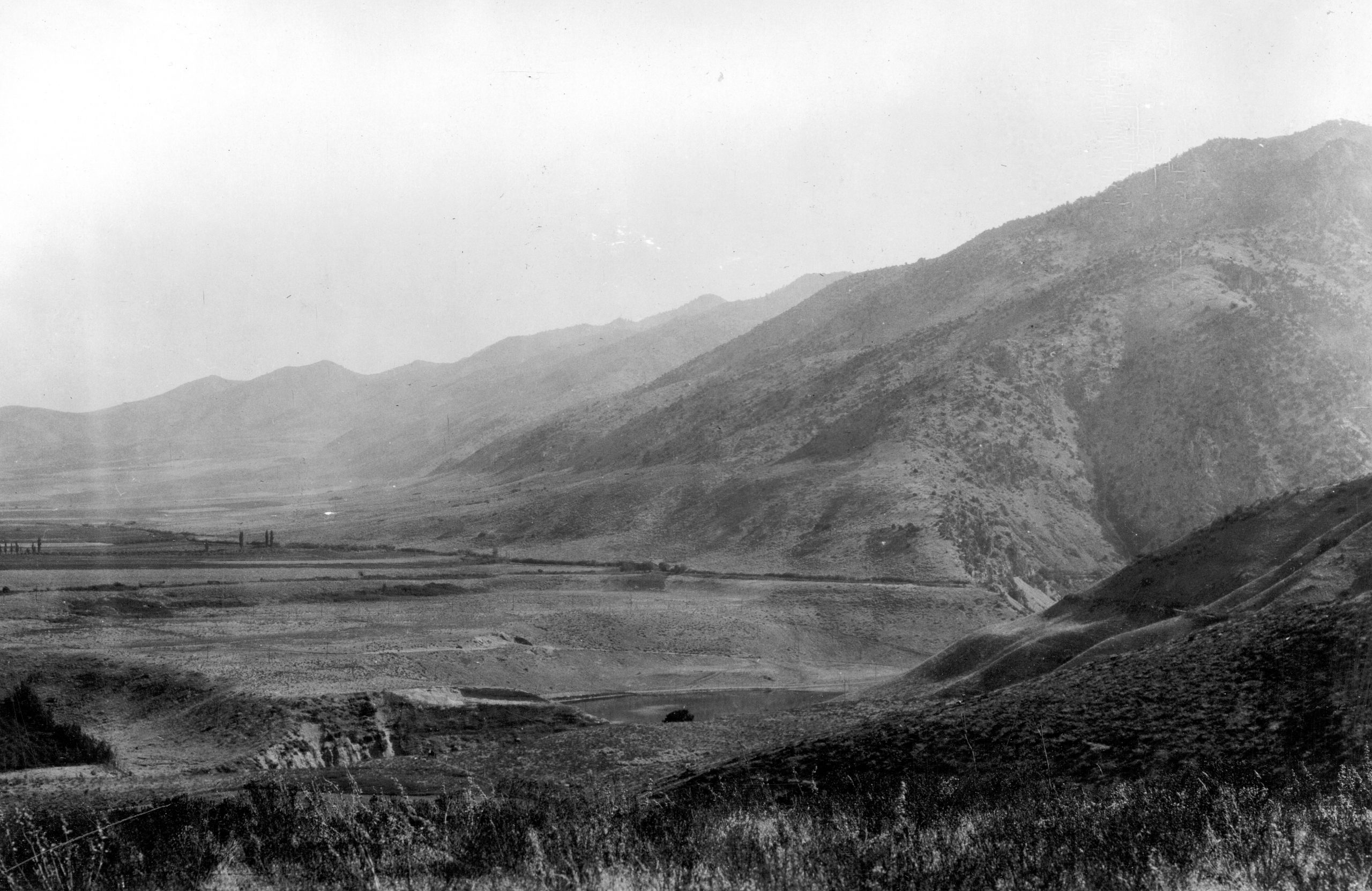
Stream terraces are remnants of older floodplains located above the existing floodplain and river. Like entrenched meanders, stream terraces form when uplift occurs or base level drops and streams erode downward, their meanders widening a new flood plain. Stream terraces can also form from extreme flood events associated with retreating glaciers. A classic example of multiple stream terraces are along the Snake River in Grand Teton National Park in Wyoming [32; 33].
![]() |
![]() |
Take this quiz to check your comprehension of this section.
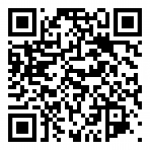
11.6 Groundwater
Groundwater is an important source of freshwater. It can be found at varying depths in all places under the ground, but is limited by extractable quantity and quality.
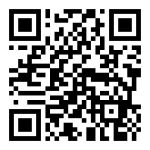
11.6.1 Porosity and Permeability
An aquifer is a rock unit that contains extractable ground water. A good aquifer must be both porous and permeable. Porosity is the space between grains that can hold water, expressed as the percentage of open space in the total volume of the rock. Permeability comes from connectivity of the spaces that allows water to move in the aquifer. Porosity can occur as primary porosity, as space between sand grains or vesicles in volcanic rocks, or secondary porosity as fractures or dissolved spaces in rock). Compaction and cementation during lithification of sediments reduces porosity (see chapter 5.3).
A combination of a place to contain water (porosity) and the ability to move water (permeability) makes a good aquifer—a rock unit or sediment that allows extraction of groundwater. Well-sorted sediments have higher porosity because there are not smaller sediment particles filling in the spaces between the larger particles. Shales made of clays generally have high porosity, but the pores are poorly connected, thereby causing low permeability.
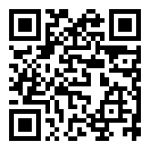
While permeability is an important measure of a porous material’s ability to transmit water, hydraulic conductivity is more commonly used by geologists to measure how easily a fluid is transmitted. Hydraulic conductivity measures both the permeability of the porous material and the properties of the water, or whatever fluid is being transmitted like oil or gas. Because hydraulic conductivity also measures the properties of the fluid, such as viscosity, it is used by both petroleum geologists and hydrogeologists to describe both the production capability of oil reservoirs and of aquifers. High hydraulic conductivity indicates that fluid transmits rapidly through an aquifer.
11.6.2 Aquifers
Aquifers are rock layers with sufficient porosity and permeability to allow water to be both contained and move within them. For rock or sediment to be considered an aquifer, its pores must be at least partially filled with water and it must be permeable enough to transmit water. Drinking water aquifers must also contain potable water. Aquifers can vary dramatically in scale, from spanning several formations covering large regions to being a local formation in a limited area. Aquifers adequate for water supply are both permeable, porous, and potable.
11.6.3 Groundwater Flow
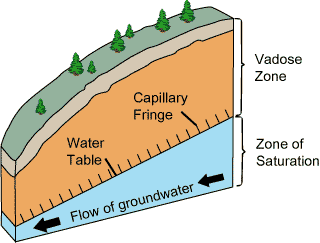
When surface water infiltrates or seeps into the ground, it usually enters the unsaturated zone also called the vadose zone, or zone of aeration. The vadose zone is the volume of geologic material between the land surface and the zone of saturation where the pore spaces are not completely filled with water [34]. Plant roots inhabit the upper vadose zone and fluid pressure in the pores is less than atmospheric pressure. Below the vadose zone is the capillary fringe. Capillary fringe is the usually thin zone below the vadose zone where the pores are completely filled with water (saturation), but the fluid pressure is less than atmospheric pressure. The pores in the capillary fringe are filled because of capillary action, which occurs because of a combination of adhesion and cohesion. Below the capillary fringe is the saturated zone or phreatic zone, where the pores are completely saturated and the fluid in the pores is at or above atmospheric pressure. The interface between the capillary fringe and the saturated zone marks the location of the water table.
Wells are conduits that extend into the ground with openings to the aquifers, to extract from, measure, and sometimes add water to the aquifer. Wells are generally the way that geologists and hydrologist measure the depth to groundwater from the land surface as well as withdraw water from aquifers.
Water is found throughout the pore spaces in sediments and bedrock. The water table is the area below which the pores are fully saturated with water. The simplest case of a water table is when the aquifer is unconfined, meaning it does not have a confining layer above it. Confining layers can pressurize aquifers by trapping water that is recharged at a higher elevation underneath the confining layer, allowing for a potentiometric surface higher than the top of the aquifer, and sometimes higher than the land surface.
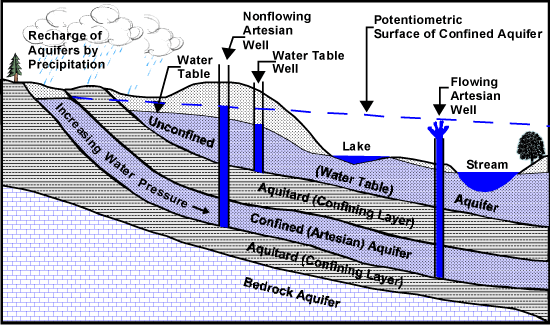
A confining layer is a low permeability layer above and/or below an aquifer that restricts the water from moving in and out of the aquifer. Confining layers include aquicludes, which are so impermeable that no water travels through them, and aquitards, which significantly decrease the speed at which water travels through them. The potentiometric surface represents the height that water would rise in a well penetrating the pressurized aquifer system. Breaches in the pressurized aquifer system, like faults or wells, can cause springs or flowing wells, also known as artesian wells.
The water table will generally mirror surface topography, though more subdued, because hydrostatic pressure is equal to atmospheric pressure along the surface of the water table. If the water table intersects the ground surface the result will be water at the surface in the form of a gaining stream, spring, lake, or wetland. The water table intersects the channel for gaining streams which then gains water from the water table. The channels for losing streams lie below the water table, thus losing streams lose water to the water table. Losing streams may be seasonal during a dry season or ephemeral in dry climates where they may normally be dry and carry water only after rain storms. Ephemeral streams pose a serious danger of flash flooding in dry climates.
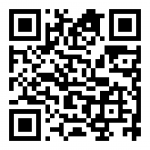
Mentioned in the video is the USGS Groundwater Watch site.
Using wells, geologists measure the water table’s height and the potentiometric surface. Graphs of the depth to groundwater over time, are known as hydrographs and show changes in the water table over time. Well-water level is controlled by many factors and can change very frequently, even every minute, seasonally, and over longer periods of time.
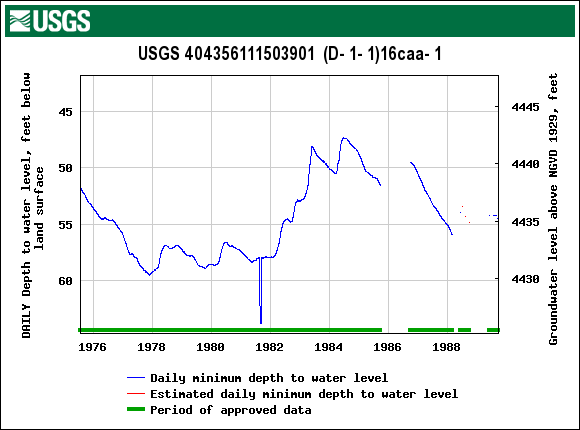
In 1856, French engineer Henry Darcy developed a hypothesis to show how discharge through a porous medium is controlled by permeability, pressure, and cross- sectional area. To prove this relationship, Darcy experimented with tubes of packed sediment with water running through them. The results of his experiments empirically established a quantitative measure of hydraulic conductivity and discharge that is known as Darcy’s law. The relationships described by Darcy’s Law have close similarities to Fourier's law in the field of heat conduction, Ohm's law in the field of electrical networks, or Fick's law in diffusion theory.
Q=KA(Δh/L)
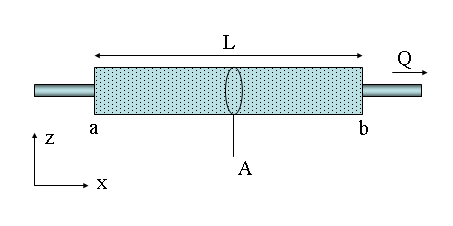
- Q = flow (volume/time)
- K = hydraulic conductivity (length/time)
- A = cross-sectional area of flow (area)
- Δh = change in pressure head (pressure difference)
- L = distance between pressure (h) measurements (length)
- Δh/L is commonly referred to as the hydraulic gradient
Pumping water from an unconfined aquifer lowers the water table. Pumping water from a confined aquifer lowers the pressure and/or potentiometric surface around the well. In an unconfined aquifer, the water table is lowered as water is removed from the aquifer near the well producing drawdown and a cone of depression (see figure). In a confined aquifer, pumping on an artesian well reduces the pressure or potentiometric surface around the well.
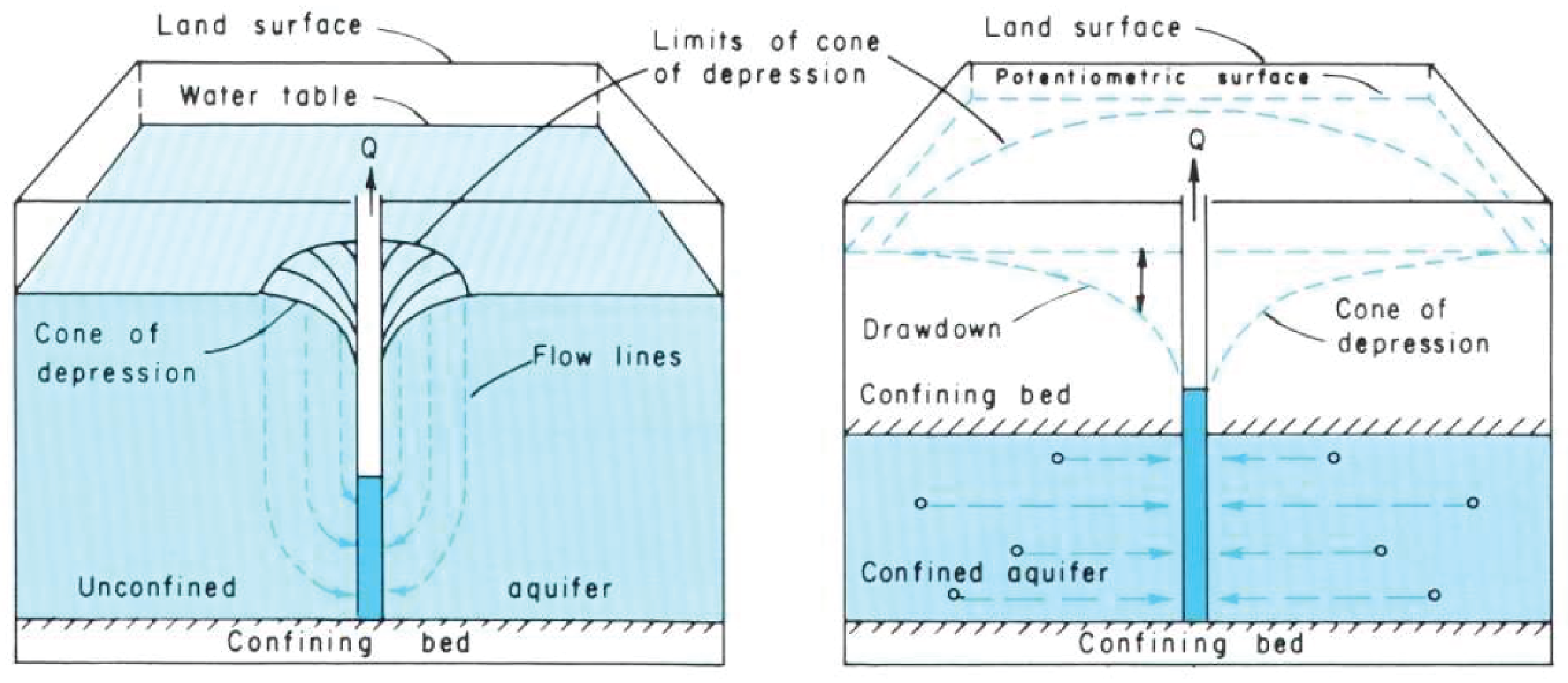
When one cone of depression intersects another cone of depression or a barrier feature like an impermeable mountain block, drawdown is intensified. When a cone of depression intersects a recharge zone, the cone of depression is lessened.
11.6.4 Recharge
The recharge area is where surface water enters an aquifer through the process of infiltration. Recharge areas are generally topographically high locations of an aquifer. They are characterized by losing streams and permeable rock that allows infiltration into the aquifer. Recharge areas mark the beginning of groundwater flow paths.
In the Basin and Range Province, recharge areas for the unconsolidated aquifers of the valleys are along mountain foothills. In the foothills of Salt Lake Valley, losing streams contribute water to the gravel-rich deltaic deposits of ancient Lake Bonneville, in some cases feeding artesian wells in the Salt Lake Valley.
An aquifer management practice is to induce recharge through storage and recovery. Geologists and hydrologists can increase the recharge rate into an aquifer system using injection wells and infiltration galleries or basins [35]. Injection wells pump water into an aquifer where it can be stored. Injection wells are regulated by state and federal governments to ensure that the injected water is not negatively impacting the quality or supply of the existing groundwater in the aquifer. Some aquifers can store significant quantities of water, allowing water managers to use the aquifer system like a surface reservoir. Water is stored in the aquifer during periods of low water demand and high water supply and later extracted during times of high water demand and low water supply.
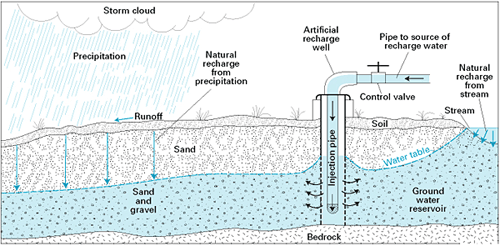
11.6.5 Discharge
Discharge areas are where the water table or potentiometric surface intersects the land surface. Discharge areas mark the end of groundwater flow paths. These areas are characterized by springs, flowing (artesian) wells, gaining streams, and playas in the dry valley basins of the Basin and Range Province of the western United States.
11.6.6 Groundwater mining and subsidence
Like other natural resources on our planet, the quantity of fresh and potable water is finite. The only natural source of water on land is from the sky in the form of precipitation. In many places, groundwater is being extracted faster than it is being replenished. When groundwater is extracted faster than it is recharged, groundwater levels and potentiometric surfaces decline, and discharge areas diminish or dry up completely. Regional pumping-induced groundwater decline is known as groundwater mining or groundwater overdraft. Groundwater mining is a serious situation and can lead to dry wells, reduced spring and stream flow, and subsidence. Groundwater mining is happening is places where more water is extracted by pumping than is being replenished by precipitation, and the water table is continually lowered. In these situations, groundwater must be viewed as a ore body and in its depletion, the possibility of producing ghost towns.
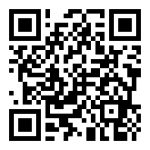
In many places, water actually helps hold up an aquifer’s skeleton by the water pressure exerted on the grains in an aquifer. This pressure is called pore pressure and comes from the weight of overlying water. If pore pressure decreases because of groundwater mining, the aquifer can compact, causing the surface of the ground to sink. Areas especially susceptible to this effect are aquifers made of unconsolidated sediments. Unconsolidated sediments with multiple layers of clay and other fine-grained material are at higher risk because when water is drained, clay compacts considerably [36; 37].
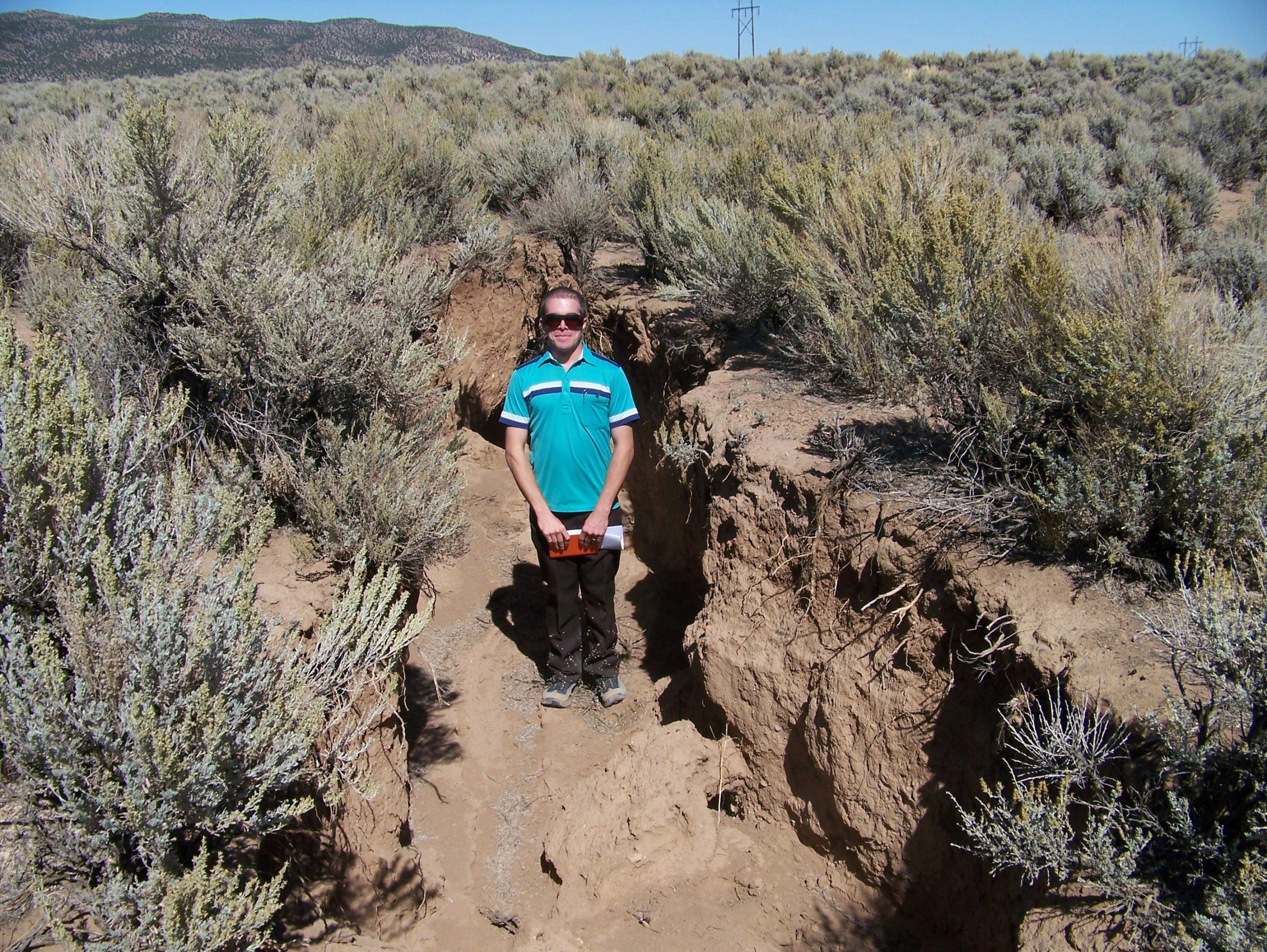
Subsidence from groundwater mining has been documented in southwestern Utah, notably Cedar Valley, Iron County, Utah. Groundwater levels have declined more than 100 feet in certain parts of Cedar Valley, causing earth fissures and measurable amounts of land subsidence.
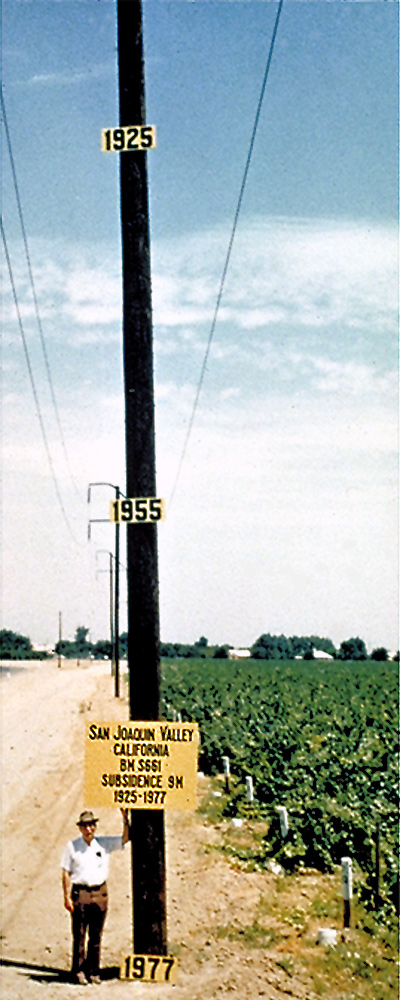
This photo shows documentation of subsidence from pumping of groundwater for irrigation in the Central Valley in California. The pole shows subsidence from groundwater pumping over a period of time.
Take this quiz to check your comprehension of this section.
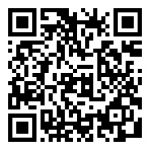
11.7 Water Contamination and Remediation
Water can be contaminated by natural features like mineral-rich geologic formations and by human activities such as agriculture, industrial operations, landfills, animal operations, and sewage treatment processes, among many other things. As water runs over the land or infiltrates into the ground, it dissolves material left behind by these potential contaminant sources. There are three major groups of contamination: organic and inorganic chemicals and biological agents. Small sediments that cloud water, causing turbidity, is also an issue with some wells, but it is not considered contamination. The risks and type of remediation for a contaminant depends on the type of chemicals present.
Contamination occurs as point-source and nonpoint-source pollution. Point source pollution can be attributed to a single, definable source, while nonpoint source pollution is from multiple dispersed sources. Point sources include waste disposal sites, storage tanks, sewage treatment plants, and chemical spills. Nonpoint sources are dispersed and indiscreet, where the whole of the contribution of pollutants is harmful, but the individual components do not have harmful concentrations of pollutants. A good example of nonpoint pollution is residential areas, where lawn fertilizer on one person’s yard may not contribute much pollution to the system, but the combined effect of many residents using fertilizer can lead to significant nonpoint pollution. Other nonpoint sources include nutrients (nitrate and phosphate), herbicides, pesticides contributed by farming, nitrate contributed by animal operations, and nitrate contributed by septic systems.
Organic chemicals are common pollutants. They consist of strands and rings of carbon atoms, usually connected by covalent bonds. Other types of atoms, like chlorine, and molecules, like hydroxide (OH-), are attached to the strands and rings. The number and arrangement of atoms will decide how the chemical behaves in the environment, its danger to humans or ecosystems, and where the chemical ends up in the environment. The different arrangements of carbon allow for tens of thousands of organic chemicals, many of which have never been studied for negative effects on human health or the environment. Common organic pollutants are herbicides and pesticides, pharmaceuticals, fuel, and industrial solvents and cleansers.
Organic chemicals include surfactants such as cleaning agents and synthetic hormones associated with pharmaceuticals, which can act as endocrine disruptors. Endocrine disruptors mimic hormones, and can cause long-term effects in developing sexual reproduction systems in developing animals. Only very small quantities of endocrine disruptors are needed to cause significant changes in animal populations.
An example of organic chemical contamination is the Love Canal, Niagara Falls, New York. From 1942 to 1952, the Hooker Chemical Company disposed of over 21,337 mt (21,000 t) of chemical waste, including chlorinated hydrocarbons, into a canal and covered it with a thin layer of clay. Chlorinated hydrocarbons are a large group of organic chemicals that have chlorine functional groups, most of which are toxic and carcinogenic to humans. The company sold the land to the New York School Board, who developed it into a neighborhood. After residents began to suffer from serious health ailments and pools of oily fluid started rising into residents’ basements, the neighborhood had to be evacuated. This site became a U.S. Environmental Protection Agency Superfund site, a site with federal funding and oversight to ensure its cleanup.
Inorganic chemicals are another set of chemical pollutants. They can contain carbon atoms, but not in long strands or links. Inorganic contaminants include chloride, arsenic, and nitrate (NO3). Nutrients can be from geologic material, like phosphorous-rich rock, but are most often sourced from fertilizer and animal and human waste. Untreated sewage and agricultural runoff contain concentrates of nitrogen and phosphorus which are essential for the growth of microorganisms. Nutrients like nitrate and phosphate in surface water can promote growth of microbes, like blue-green algae (cyanobacteria), which in turn use oxygen and create toxins (microcystins and anatoxins) in lakes [38]. This process is known as eutrophication.
Metals are common inorganic contaminants. Lead, mercury, and arsenic are some of the more problematic inorganic groundwater contaminants. Bangladesh has a well documented case of arsenic contamination from natural geologic material dissolving into the groundwater. Acid-mine drainage can also cause significant inorganic contamination (see Chapter 16).
Salt, typically sodium chloride, is a common inorganic contaminant. It can be introduced into groundwater from natural sources, such as evaporite deposits like the Arapien Shale of Utah, or from anthropogenic sources like the salts applied to roads in the winter to keep ice from forming. Salt contamination can also occur near ocean coasts from saltwater intruding into the cones of depression around fresh groundwater pumping, inducing the encroachment of saltwater into the freshwater body.
Biological agents are another common groundwater contaminant which includes harmful bacteria and viruses. A common bacteria contaminant is Escherichia coli (E. coli). Generally, harmful bacteria are not present in groundwater unless the groundwater source is closely connected with a contaminated surface source, such as a septic system. Karst, landforms created from dissolved limestone, is especially susceptible to this form of contamination, because water moves relatively quickly through the conduits of dissolved limestone. Bacteria can also be used for remediation.
Table. Groundwater contaminants.
Remediation is the act of cleaning contamination. Hydrologists use three types of remediation: biological, chemical, and physical. Biological remediation uses specific strains of bacteria to break down a contaminant into safer chemicals. This type of remediation is usually used on organic chemicals, but also works on reducing or oxidizing inorganic chemicals like nitrate. Phytoremediation is a type of bioremediation that uses plants to absorb the chemicals over time.
Chemical remediation uses chemicals to remove the contaminant or make it less harmful. One example is to use a reactive barrier, a permeable wall in the ground or at a discharge point that chemically reacts with contaminants in the water. Reactive barriers made of limestone can increase the pH of acid mine drainage, making the water less acidic and more basic, which removes dissolved contaminants by precipitation into solid form.
Physical remediation consists of removing the contaminated water and either treating it with filtration, called pump-and-treat, or disposing of it. All of these options are technically complex, expensive, and difficult, with physical remediation typically being the most costly.
Take this quiz to check your comprehension of this section.
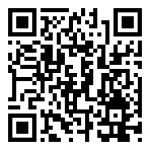
11.8 Karst
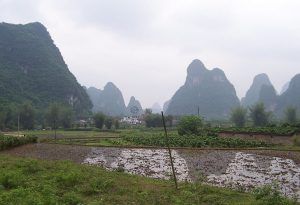
Karst refers to landscapes and hydrologic features created by the dissolving of limestone. Karst can be found anywhere there is limestone and other soluble subterranean substances like salt deposits. Dissolving of limestone creates features like sinkholes, caverns, disappearing streams, and towers.
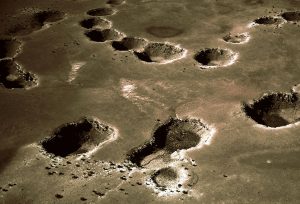
Dissolving of underlying salt deposits has caused sinkholes to form in the Kaibab Limestone on the Colorado Plateau in Arizona.
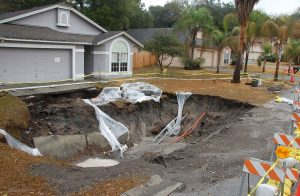
Collapse of the surface into an underground cavern caused this sinkhole in the front yard of a home in Florida.
CO2 in the atmosphere dissolves readily in the water droplets that form clouds from which precipitation comes in the form of rain and snow. This precipitation is slightly acidic with carbonic acid. Karst forms when carbonic acid dissolves calcite (calcium carbonate) in limestone.
H2O + CO2 = H2CO3
Water + Carbon Dioxide Gas equals Carbonic Acid in Water
CaCO3 + H2CO3 = Ca2++ 2HCO3 -1
Solid Calcite + Carbonic Acid in Water Dissolved equals Calcium Ion + Dissolved Bicarbonate Ion
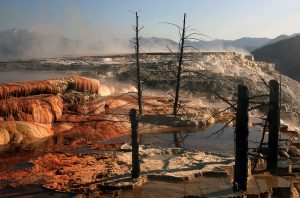
After the slightly acidic water dissolves the calcite, changes in temperature or gas content in the water can cause the water to redeposit the calcite in a different place as tufa (travertine), often deposited by a spring or in a cave. Speleothems are secondary deposits, typically made of travertine, deposited in a cave. Travertine speleothems form by water dripping through cracks and dissolved openings in caves and evaporating, leaving behind the travertine deposits. Speleothems commonly occur in the form of stalactites, when extending from the ceiling, and stalagmites, when standing up from the floor.
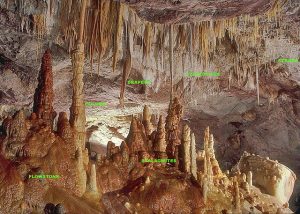
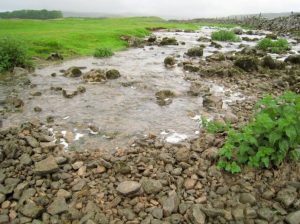
Surface water enters the karst system through sinkholes, losing streams, and disappearing streams. Changes in base level can cause rivers running over limestone to dissolve the limestone and sink into the ground. As the water continues to dissolve its way through the limestone, it can leave behind intricate networks of caves and narrow passages. Often dissolution will follow and expand fractures in the limestone. Water exits the karst system as springs and rises. In mountainous terrane, dissolution can extend all the way through the vertical profile of the mountain, with caverns dropping thousands of feet.
Take this quiz to check your comprehension of this section.
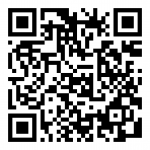
Summary
Water is essential for all living things. It continuously cycles through the atmosphere, over land, and through the ground. In much of the United States and other countries, water is managed through a system of regional laws and regulations and distributed on paper in a system collectively known as “water rights”. Surface water follows a watershed, which is separate from other areas by its divides (highest ridges). Groundwater exists in the pores within rocks and sediment. It moves predominantly due to pressure and gravitational gradients through the rock. Human and natural causes can make water unsuitable for consumption. There are different ways to deal with this contamination. Karst is when limestone is dissolved by water, forming caves and sinkholes.
Take this quiz to check your comprehension of this Chapter.
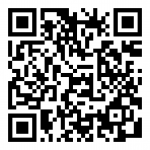
References
- Brush, L.M., Jr, 1961, Drainage basins, channels, and flow characteristics of selected streams in central Pennsylvania: pubs.er.usgs.gov.
- Charlton, R., 2007, Fundamentals of fluvial geomorphology: Taylor & Francis.
- Cirrus Ecological Solutions, 2009, Jordan River TMDL: Utah State Division of Water Quality.
- Earle, S., 2015, Physical geology OER textbook: BC Campus OpenEd.
- EPA, 2009, Water on Tap-What You Need to Know: U.S. Environmental Protection Agency.
- Fagan, B., 2012, Elixir: A history of water and humankind: Bloomsbury Press.
- Fairbridge, R.W., 1968, Yazoo rivers or streams, in Geomorphology: Springer Berlin Heidelberg Encyclopedia of Earth Science, p. 1238–1239.
- Freeze, A.R., and Cherry, J.A., 1979, Groundwater: Prentice Hall.
- Galloway, D., Jones, D.R., and Ingebritsen, S.E., 1999, Land subsidence in the United States: U.S. Geological Survey Circular 1182.
- Galloway, W.E., Whiteaker, T.L., and Ganey-Curry, P., 2011, History of Cenozoic North American drainage basin evolution, sediment yield, and accumulation in the Gulf of Mexico basin: Geosphere, v. 7, no. 4, p. 938–973.
- Gilbert, G.K., 1890, Lake Bonneville: United States Geological Survey, 438 p.
- Gleick, P.H., 1993, Water in Crisis: A Guide to the World’s Fresh Water Resources: Oxford University Press.
- Hadley, G., 1735, Concerning the cause of the general trade-winds: By Geo. Hadley, Esq; FRS: Philosophical Transactions, v. 39, no. 436–444, p. 58–62.
- Halvorson, S.F., and James Steenburgh, W., 1999, Climatology of lake-effect snowstorms of the Great Salt Lake: University of Utah.
- Heath, R.C., 1983, Basic ground-water hydrology: U.S. Geological Survey Water-Supply Paper 2220, 91 p.
- Hobbs, W.H., and Fisk, H.N., 1947, Geological Investigation of the Alluvial Valley of the Lower Mississippi River: JSTOR.
- Knudsen, T., Inkenbrandt, P., Lund, W., Lowe, M., and Bowman, S., 2014, Investigation of land subsidence and earth fissures in Cedar Valley, Iron County, Utah: Utah Geological Survey Special Study 150.
- Lorenz, E.N., 1955, Available potential energy and the maintenance of the general circulation: Tell’Us, v. 7, no. 2, p. 157–167.
- Marston, R.A., Mills, J.D., Wrazien, D.R., Bassett, B., and Splinter, D.K., 2005, Effects of Jackson lake dam on the Snake River and its floodplain, Grand Teton National Park, Wyoming, USA: Geomorphology, v. 71, no. 1–2, p. 79–98.
- Maupin, M.A., Kenny, J.F., Hutson, S.S., Lovelace, J.K., Barber, N.L., and Linsey, K.S., 2014, Estimated use of water in the United States in 2010: US Geological Survey.
- Myers, W.B., and Hamilton, W., 1964, The Hebgen Lake, Montana, earthquake of August 17, 1959: U.S. Geol. Surv. Prof. Pap., v. 435, p. 51.
- Oviatt, C.G., 2015, Chronology of Lake Bonneville, 30,000 to 10,000 yr B.P: Quat. Sci. Rev., v. 110, p. 166–171.
- Powell, J.W., 1879, Report on the lands of the arid region of the United States with a more detailed account of the land of Utah with maps: Monograph.
- Reed, J.C., Love, D., and Pierce, K., 2003, Creation of the Teton landscape: a geologic chronicle of Jackson Hole and the Teton Range: pubs.er.usgs.gov.
- Reese, R.S., 2014, Review of Aquifer Storage and Recovery in the Floridan Aquifer System of Southern Florida:
- Schele, L., Miller, M.E., Kerr, J., Coe, M.D., and Sano, E.J., 1992, The Blood of Kings: Dynasty and Ritual in Maya Art: George Braziller Inc.
- Seaber, P.R., Kapinos, F.P., and Knapp, G.L., 1987, Hydrologic unit maps:
- Solomon, S., 2011, Water: The Epic Struggle for Wealth, Power, and Civilization: Harper Perennial.
- Törnqvist, T.E., Wallace, D.J., Storms, J.E.A., Wallinga, J., Van Dam, R.L., Blaauw, M., Derksen, M.S., Klerks, C.J.W., Meijneken, C., and Snijders, E.M.A., 2008, Mississippi Delta subsidence primarily caused by compaction of Holocene strata: Nat. Geosci., v. 1, no. 3, p. 173–176.
- Turner, R.E., and Rabalais, N.N., 1991, Changes in Mississippi River water quality this century: Bioscience, v. 41, no. 3, p. 140–147.
- United States Geological Survey, 1967, The Amazon: Measuring a Mighty River: United States Geological Survey O-245-247.
- U.S. Environmental Protection Agency, 2014, Cyanobacteria/Cyanotoxins
- U.S. Geological Survey, 2012, Snowmelt - The Water Cycle, from USGS Water-Science School
- Utah/Nevada Draft Snake Valley Agreement, 2013
A dark liquid fossil fuel derived from petroleum.
Oil which is found in low-permeability, high-porosity rocks such as shale.
Gaseous fossil fuel derived from petroleum, mostly made of methane.
Rocks which allow petroleum resources to collect or move.
A rock that contains material which can be turned into petroleum resources. Organic-rich muds form good source rocks.
A geologic circumstance (such as a fold, fault, change in lithology, etc.) which allows petroleum resources to collect.
Sands or sandstones that contain high-viscosity petroleum.
Component of the gravitational force which pushes material downslope.
By McKay Savage from London, UK [CC BY 2.0], via Wikimedia Commons
QR Code generated with QRCode Monkey. All generated QR Codes are 100% free and can be used for whatever you want. This includes all commercial purposes.
Large metallic mineral deposit that forms near magma bodies like plutons. Commonly contains copper, lead, zinc, molybdenum, and gold.
Carbonate rock that reacts with hot magmatic fluids, creating concentrated ore deposits, which include copper, iron, zinc, and gold.
QR Code generated with QRCode Monkey. All generated QR Codes are 100% free and can be used for whatever you want. This includes all commercial purposes.
Lowest layer of the soil (C), which is mechanically weathered (not chemically weathered) bedrock.
Rob Lavinsky, iRocks.com – CC-BY-SA-3.0 [CC BY-SA 3.0], via Wikimedia Commons
By Dave Bunnell / Under Earth Images (Own work) [CC BY-SA 2.5], via Wikimedia Commons
A rule that says the outer valence shell of electrons is complete when it contains 8 electrons.
QR Code generated with QRCode Monkey. All generated QR Codes are 100% free and can be used for whatever you want. This includes all commercial purposes.
Smooth surface carved in harder rocks by glacial action.
Dunes that form semicircular shapes due to anchoring vegetation.
By Matt Affolter(QFL247) (talk) (Transferred by Citypeek/Original uploaded by Matt Affolter(QFL247)) [CC BY-SA 3.0 or GFDL], via Wikimedia Commons
https://waterdata.usgs.gov/nwis/dv/?ts_id=143976&format=img_default&site_no=404356111503901&set_arithscale_y=on&begin_date=19750718&end_date=19890930
QR Code generated with QRCode Monkey. All generated QR Codes are 100% free and can be used for whatever you want. This includes all commercial purposes.
By U.S. Geological Survey from Reston, VA, USA (Sinkholes) [CC0 or Public domain], via Wikimedia Commons
By Amcyrus2012 (Own work) [CC BY 4.0], via Wikimedia Commons
QR Code generated with QRCode Monkey. All generated QR Codes are 100% free and can be used for whatever you want. This includes all commercial purposes.
A rock made of primarily silt.
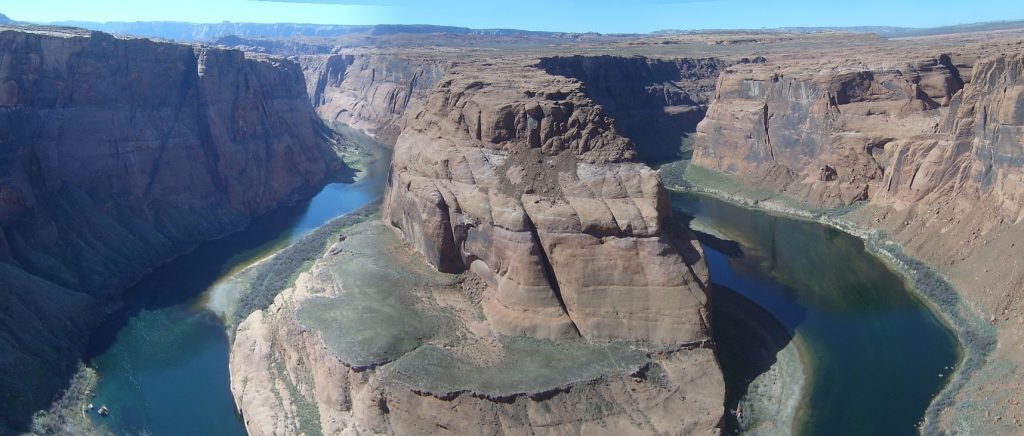
11 Water
KEY CONCEPTS
-
Example of a Roman aqueduct in Segovia, Spain. Describe the processes of the water cycle
- Describe drainage basins, watershed protection, and water budget
- Describe reasons for water laws, who controls them, and how water is shared in the western U.S.
- Describe zone of transport, zone of sediment production, zone of deposition, and equilibrium
- Describe stream landforms: channel types, alluvial fans, floodplains, natural levees, deltas, entrenched meanders, and terraces
- Describe the properties required for a good aquifer; define confining layer water table
- Describe three major groups of water contamination and three types of remediation
- Describe karst topography, how it is created, and the landforms that characterize it
All life on Earth requires water. The hydrosphere (Earth’s water) is an important agent of geologic change. Water shapes our planet by depositing minerals, aiding lithification, and altering rocks after they are lithified. Water carried by subducted oceanic plates causes flux melting of upper mantle material. Water is among the volatiles in magma and emerges at the surface as steam in volcanoes.
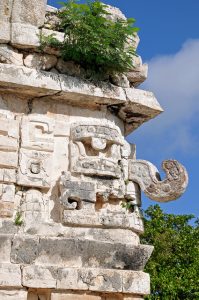
Humans rely on suitable water sources for consumption, agriculture, power generation, and many other purposes. In pre-industrial civilizations, the powerful controlled water resources [1, 2]. As shown in the figures, two thousand year old Roman aqueducts still grace European, Middle Eastern, and North African skylines. Ancient Mayan architecture depicts water imagery such as frogs, water-lilies, water fowl to illustrate the importance of water in their societies [3]. In the drier lowlands of the Yucatan Peninsula, mask facades of the hooked-nosed rain god, Chac (or Chaac) are prominent on Mayan buildings such as the Kodz Poop (Temple of the Masks, sometimes spelled Coodz Poop) at the ceremonial site of Kabah. To this day government controlled water continues to be an integral part of most modern societies.
11.1 Water Cycle
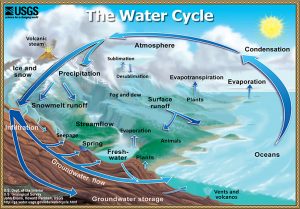
The water cycle is the continuous circulation of water in the Earth's atmosphere. During circulation, water changes between solid, liquid, and gas (water vapor) and changes location. The processes involved in the water cycle are evaporation, transpiration, condensation, precipitation, and runoff.
Evaporation is the process by which a liquid is converted to a gas. Water evaporates when solar energy warms the water sufficiently to excite the water molecules to the point of vaporization. Evaporation occurs from oceans, lakes, and streams and the land surface. Plants contribute significant amounts of water vapor as a byproduct of photosynthesis called transpiration that occurs through the minute pores of plant leaves. The term evapotranspiration refers to these two sources of water entering the atmosphere and is commonly used by geologists.
Water vapor is invisible. Condensation is the process of water vapor transitioning to a liquid. Winds carry water vapor in the atmosphere long distances. When water vapor cools or when air masses of different temperatures mix, water vapor may condense back into droplets of liquid water. These water droplets usually form around a microscopic piece of dust or salt called condensation nuclei. These small droplets of liquid water suspended in the atmosphere become visible as in a cloud. Water droplets inside clouds collide and stick together, growing into larger droplets. Once the water droplets become big enough, they fall to Earth as rain, snow, hail, or sleet.
Once precipitation has reached the Earth's surface, it can evaporate or flow as runoff into streams, lakes, and eventually back to the oceans. Water in streams and lakes is called surface water. Or water can also infiltrate into the soil and fill the pore spaces in the rock or sediment underground to become groundwater. Groundwater slowly moves through rock and unconsolidated materials. Some groundwater may reach the surface again, where it discharges as springs, streams, lakes, and the ocean. Also, surface water in streams and lakes can infiltrate again to recharge groundwater. Therefore, the surface water and groundwater systems are connected.
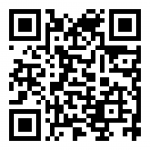
Take this quiz to check your comprehension of this section.
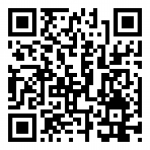
11.2 Water Basins and Budgets
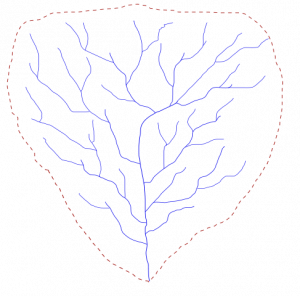
The basic unit of division of the landscape is the drainage basin, also known as a catchment or watershed. It is the area of land that captures precipitation and contributes runoff to a stream or stream segment [4]. Drainage divides are local topographic high points that separate one drainage basin from another [5]. Water that falls on one side of the divide goes to one stream, and water that falls on the other side of the divide goes to a different stream. Each stream, tributary and streamlet has its own drainage basin. In areas with flatter topography, drainage divides are not as easily identified but they still exist [6].
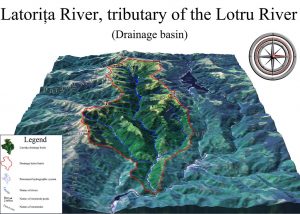
The headwater is where the stream begins. Smaller tributary streams combine downhill to make the larger trunk of the stream. The mouth is where the stream finally reaches its end. The mouth of most streams is at the ocean. However, a rare number of streams do not flow to the ocean, but rather end in a closed basin (or endorheic basin) where the only outlet is evaporation. Most streams in the Great Basin of Western North America end in endorheic basins. For example, in Salt Lake County, Utah, Little Cottonwood Creek and the Jordan River flow into the endorheic Great Salt Lake where the water evaporates.
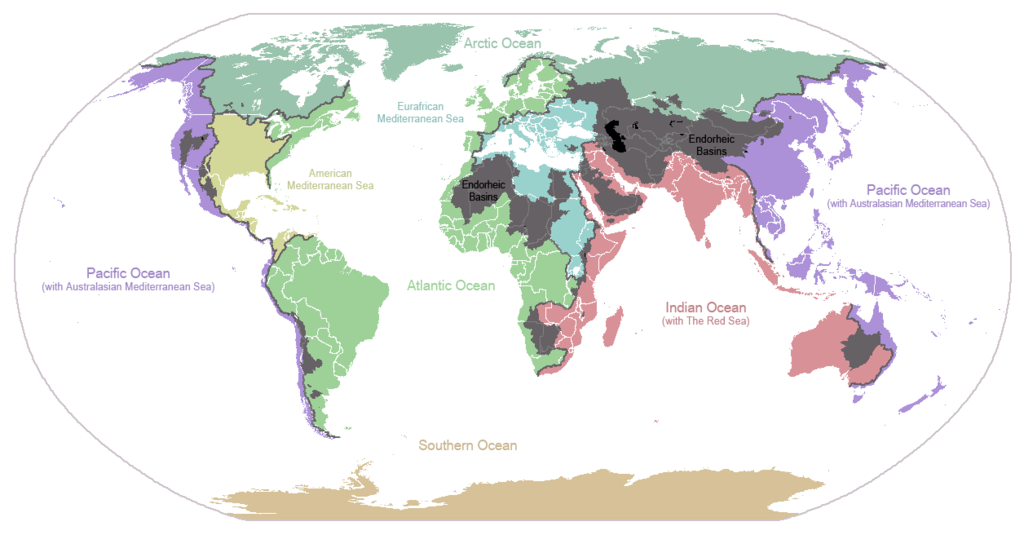
Perennial streams flow all year round. Perennial streams occur in humid or temperate climates where there is sufficient rainfall and low evaporation rates. Water levels rise and fall with the seasons, depending on the discharge. Ephemeral streams flow only during rain events or the wet season. In arid climates, like Utah, many streams are ephemeral. These streams occur in dry climates with low amounts of rainfall and high evaporation rates. Their channels are often dry washes or arroyos for much of the year and their sudden flow causes flash floods [7].
Along Utah’s Wasatch Front, the urban area extending north to south from Brigham City to Provo, there are several watersheds that are designated as “watershed protection areas” that limit the type of use allowed in those drainages in order to protect culinary water. Dogs and swimming are limited in those watersheds because of the possibility of contamination by harmful bacteria and substances to the drinking supply of Salt Lake City and surrounding municipalities.
Water in the water cycle is very much like money in a personal budget. Income includes precipitation and stream and groundwater inflow. Expenses include groundwater withdrawal, evaporation, and stream and groundwater outflow. If the expenses outweigh the income, the water budget is not balanced. In this case, water is removed from savings, i.e. water storage, if available. Reservoirs, snow, ice, soil moisture, and aquifers all serve as storage in a water budget. In dry regions, the water is critical for sustaining human activities. Understanding and managing the water budget is an ongoing political and social challenge.
Hydrologists create groundwater budgets within any designated area, but they are generally made for watershed (basin) boundaries, because groundwater and surface water are easier to account for within these boundaries. Water budgets can be created for state, county, or aquifer extent boundaries as well. The groundwater budget is an essential component of the hydrologic model; hydrologists use measured data with a conceptual workflow of the model to better understand the water system.
Take this quiz to check your comprehension of this section.
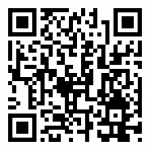
11.3 Water Use and Distribution
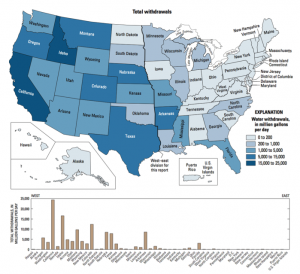
In the United States, 1,344 billion L (355 billion gallons) of ground and surface water are used each day, of which 288 billion L (76 billion gallons) are fresh groundwater. The state of California uses 16% of national groundwater [8].
Utah is the second driest state in the United States. Nevada, having a mean statewide precipitation of 31 cm (12.2 inches) per year, is the driest. Utah also has the second highest per capita rate of total domestic water use of 632.16 L (167 gallonsL per day per person [8]. With the combination of relatively high demand and limited quantity, Utah is at risk for water budget deficits.
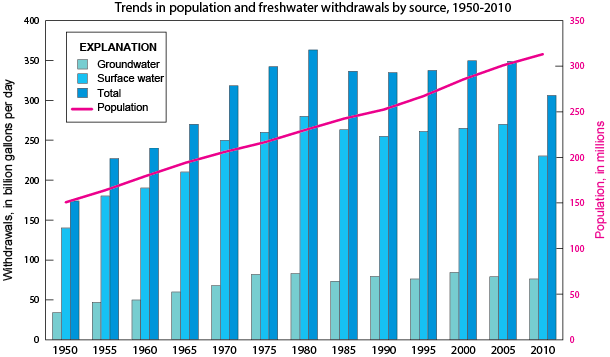
11.3.1 Surface Water Distribution
Fresh water is a precious resource and should not be taken for granted, especially in dry climates. Surface water makes up only 1.2% of the fresh water available on the planet, and 69% of that surface water is trapped in ground ice and permafrost. Stream water accounts for only 0.006% of all freshwater and lakes contain only 0.26% of the world’s fresh water [9].
Global circulation patterns are the most important factor in distributing surface water through precipitation. Due to the Coriolis effect and the uneven heating of the Earth, air rises near the equator and near latitudes 60° north and south. Air sinks at the poles and latitudes 30° north and south (see Chapter 13). Land masses near rising air are more prone to humid and wet climates. Land masses near sinking air, which inhibits precipitation, are prone to dry conditions [10, 11]. Prevailing winds, ocean circulation patterns such as the Gulf Stream’s effects on eastern North America, rain shadows (the dry leeward sides of mountains), and even the proximity of bodies of water can affect local climate patterns. When this moist air collides with the nearby mountains causing it to rise and cool, the moisture may fall out as snow or rain on nearby areas in a phenomenon known as “lake-effect precipitation.” [12]
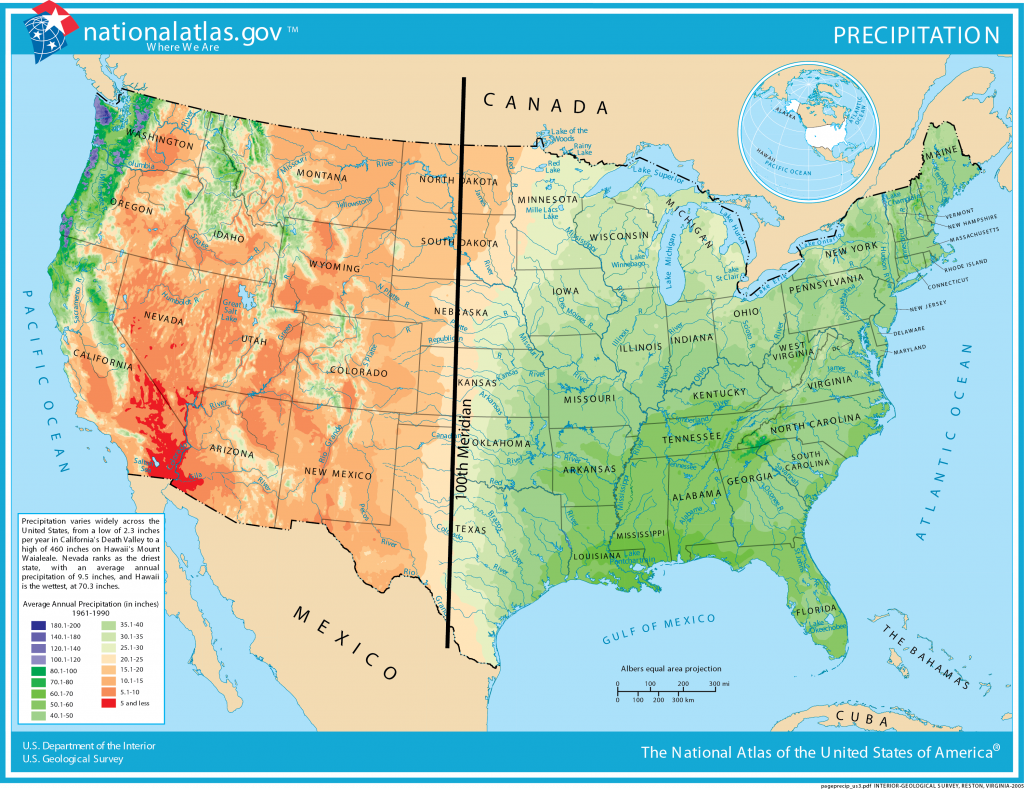
In the United States, the 100th meridian roughly marks the boundary between the humid and arid parts of the country. Growing crops west of the 100th meridian requires irrigation [13]. In the west, surface water is stored in reservoirs and mountain snowpacks [14], then strategically released through a system of canals during times of high water use.
Some of the driest parts of the western United States are in the Basin and Range Province. The Basin and Range has multiple mountain ranges that are oriented north to south. Most of the basin valleys in the Basin and Range are dry, receiving less than 30 cm (12 inches) of precipitation per year. However, some of the mountain ranges can receive more than 1.52 m (60 inches) of water as snow or snow-water-equivalent. The snow-water equivalent is the amount of water that would result if the snow were melted, as the snowpack is generally much thicker than the equivalent amount of water that it would produce [12].
11.3.2 Groundwater Distribution
Water source | Water volume
(cubic miles) |
Fresh water (%) | Total water (%) |
---|---|---|---|
Oceans, Seas, & Bays | 321,000,000 | -- | 96.5 |
Ice caps, Glaciers, & Permanent Snow | 5,773,000 | 68.7 | 1.74 |
Groundwater | 5,614,000 | -- | 1.69 |
-- Fresh | 2,526,000 | 30.1 | 0.76 |
-- Saline | 3,088,000 | -- | 0.93 |
Soil Moisture | 3,959 | 0.05 | 0.001 |
Ground Ice & Permafrost | 71,970 | 0.86 | 0.022 |
Lakes | 42,320 | -- | 0.013 |
-- Fresh | 21,830 | 0.26 | 0.007 |
-- Saline | 20,490 | -- | 0.006 |
Atmosphere | 3,095 | 0.04 | 0.001 |
Swamp Water | 2,752 | 0.03 | 0.0008 |
Rivers | 509 | 0.006 | 0.0002 |
Biological Water | 269 | 0.003 | 0.0001 |
Source: Igor Shiklomanov's chapter "World fresh water resources" in Peter H. Gleick (editor), 1993, Water in Crisis: A Guide to the World's Fresh Water Resources (Oxford University Press, New York)[zotpressInText item="{P7VGIQT4}" format="%num%" brackets="yes" separator="comma"] |
Groundwater makes up 30.1% of the fresh water on the planet, making it the most abundant reservoir of fresh water accessible to most humans. The majority of freshwater, 68.7%, is stored in glaciers and ice caps as ice [9]. As the glaciers and ice caps melt due to global warming, this fresh water is lost as it flows into the oceans.
Take this quiz to check your comprehension of this section.
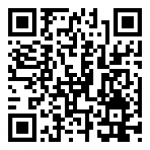
11.4 Water Law
Federal and state governments have put laws in place to ensure the fair and equitable use of water. In the United States, the states are tasked with creating a fair and legal system for sharing water.
11.4.1 Water Rights
Because of the limited supply of water, especially in the western United States, states disperse a system of legal water rights defined as a claim to a portion or all of a water source, such as a spring, stream, well, or lake. Federal law mandates that states control water rights, with the special exception of federally reserved water rights, such as those associated with national parks and Native American tribes, and navigation servitude that maintains navigable water bodies. Each state in the United States has a different way to disperse and manage water rights.
A person, entity, company, or organization, must have a water right to legally extract or use surface or groundwater in their state. Water rights in some western states are dictated by the concept of prior appropriation, or “first in time, first in right,” where the person with the oldest water right gets priority water use during times when there is not enough water to fulfill every water right.
The Colorado River and its tributaries pass through a desert region, including seven states (Wyoming, Colorado, Utah, New Mexico, Arizona, Nevada, California), Native American reservations, and Mexico. As the western United States became more populated and while California was becoming a key agricultural producer, the states along the Colorado River realized that the river was important to sustaining life in the West.
To guarantee certain perceived water rights, these western states recognized that a water budget was necesary for the Colorado River Basin. Thus was enacted the Colorado River Compact in 1922 to ensure that each state got a fair share of the river water. The Compact granted each state a specific volume of water based on the total measured flow at the time. However, in 1922, the flow of the river was higher than its long-term average flow, consequently, more water was allocated to each state than is typically available in the river [16].
Over the next several decades, lawmakers have made many other agreements and modifications regarding the Colorado River Compact, including those agreements that brought about the Hoover Dam (formerly Boulder Dam), and Glen Canyon Dam, and a treaty between the American and Mexican governments. Collectively, the agreements are referred to as “The Law of the River" by the United States Bureau of Reclamation. Despite adjustments to the Colorado River Compact, many believe that the Colorado River is still over-allocated, as the Colorado River flow no longer reaches the Pacific Ocean, its original terminus (base level). Dams along the Colorado River have caused water to divert and evaporate, creating serious water budget concerns in the Colorado River Basin. Predicted drought associated with global warming is causing additional concerns about over-allocating the Colorado River flow in the future.
The Law of the River highlights the complex and prolonged nature of interstate water rights agreements, as well as the importance of water.
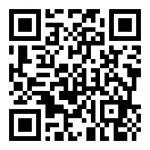
The Snake Valley straddles the border of Utah and Nevada with more of the irrigable land area lying on the Utah side of the border. In 1989, the Southern Nevada Water Authority (SNWA) submitted applications for water rights to pipe up to 191,189,707 cu m (155,000 ac-ft) of water per year (an acre-foot of water is one acre covered with water one foot deep) from Spring, Snake, Delamar, Dry Lake, and Cave valleys to southern Nevada, mostly for Las Vegas [17]. Nevada and Utah have attempted a comprehensive agreement, but negotiations have not yet been settled.
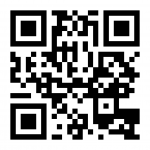
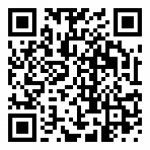
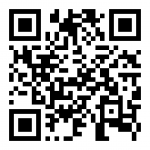
11.4.2 Water Quality and Protection
Two major federal laws that protect water quality in the United States are the Clean Water Act and the Safe Drinking Water Act. The Clean Water Act, an amendment of the Federal Water Pollution Control Act, protects navigable waters from dumping and point-source pollution. The Safe Drinking Water Act ensures that water that is provided by public water suppliers, like cities and towns, is safe to drink [18].
The U.S. Environmental Protection Agency Superfund program ensures the cleanup of hazardous contamination, and can be applied to situations of surface water and groundwater contamination. It is part of the Comprehensive Environmental Response, Compensation, and Liability Act of 1980. Under this act, state governments and the U.S. Environmental Protection Agency can use the superfund to pay for remediation of a contaminated site and then file a lawsuit against the polluter to recoup the costs. Or to avoid being sued, the polluter that caused the contamination may take direct action or provide funds to remediate the contamination.
Take this quiz to check your comprehension of this section.
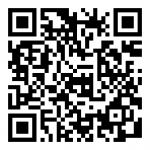
11.5 Surface Water
Geologically, a stream is a body of flowing surface water confined to a channel. Terms such as river, creek and brook are social terms not used in geology. Streams erode and transport sediments, making them the most important agents of the earth’s surface, along with wave action (see Chapter 12) in eroding and transporting sediments. They create much of the surface topography and are an important water resource.
Several factors cause streams to erode and transport sediment, but the two main factors are stream-channel gradient and velocity. Stream-channel gradient is the slope of the stream usually expressed in meters per kilometer or feet per mile. A steeper channel gradient promotes erosion. When tectonic forces elevate a mountain, the stream gradient increases, causing the mountain stream to erode downward and deepen its channel eventually forming a valley. Stream-channel velocity is the speed at which channel water flows. Factors affecting channel velocity include channel gradient which decreases downstream, discharge and channel size which increase as tributaries coalesce, and channel roughness which decreases as sediment lining the channel walls decreases in size thus reducing friction. The combined effect of these factors is that channel velocity actually increases from mountain brooks to the mouth of the stream.
11.5.1 Discharge
Stream size is measured in terms of discharge, the volume of water flowing past a point in the stream over a defined time interval. Volume is commonly measured in cubic units (length x width x depth), shown as feet3 (ft3) or meter3 (m3). Therefore, the units of discharge are cubic feet per second (ft3/sec or cfs). Therefore, the units of discharge are cubic meters per second, (m³/s or cms, or cubic feet per second (ft³/sec or cfs). Stream discharge increases downstream. Smaller streams have less discharge than larger streams. For example, the Mississippi River is the largest river in North America, with an average flow of about 16,990.11 cms (600,000 cfs) [19]. For comparison, the average discharge of the Jordan River at Utah Lake is about 16.25 cms (574 cfs) [20] and for the annual discharge of the Amazon River, (the world’s largest river), annual discharge is about 175,565 cms (6,200,000 cfs) [21].
Discharge can be expressed by the following equation:
Q = V A
- Q = discharge cms (or ft3/sec),
- A = cross-sectional area of the stream channel [width times average depth] as m2 (or in2 or ft2),
- V = average channel velocity m/s (or ft/sec) [7]
At a given location along the stream, velocity varies with stream width, shape, and depth within the stream channel as well. When the stream channel narrows but discharge remains constant, the same volume of water must flows through a narrower space causing the velocity to increase, similar to putting a thumb over the end of a backyard water hose. In addition, during rain storms or heavy snow melt, runoff increases, which increases stream discharge and velocity.
When the stream channel curves, the highest velocity will be on the outside of the bend. When the stream channel is straight and uniformly deep, the highest velocity is in the channel center at the top of the water where it is the farthest from frictional contact with the stream channel bottom and sides. In hydrology, the thalweg of a river is the line drawn that shows its natural progression and deepest channel, as is shown in the diagram.
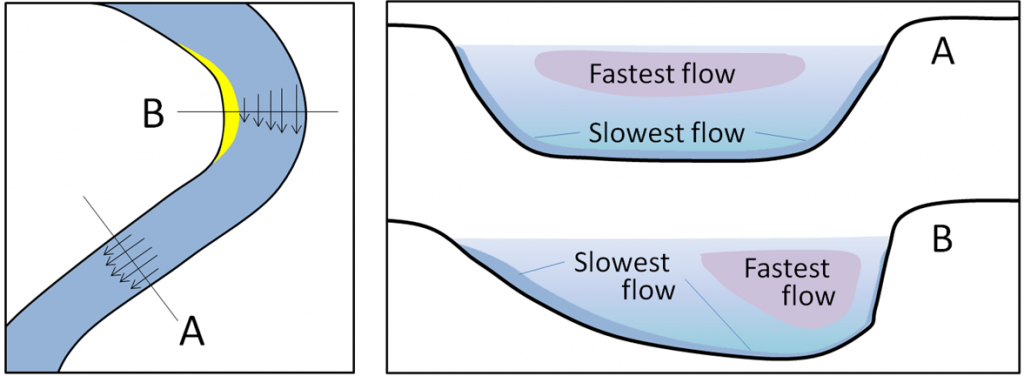
11.5.2 Runoff vs. Infiltration
Factors that dictate whether water will infiltrate into the ground or run off over the land include the amount, type, and intensity of precipitation; the type and amount of vegetation cover; the slope of the land; the temperature and aspect of the land; preexisting conditions; and the type of soil in the infiltrated area. High- intensity rain will cause more runoff than the same amount of rain spread out over a longer duration. If the rain falls faster than the soil’s properties allow it to infiltrate, then the water that cannot infiltrate becomes runoff. Dense vegetation can increase infiltration, as the vegetative cover slows the water particle’s overland flow giving them more time to infiltrate. If a parcel of land has more direct solar radiation or higher seasonal temperatures, there will be less infiltration and runoff, as evapotranspiration rates will be higher. As the land’s slope increases, so does runoff, because the water is more inclined to move downslope than infiltrate into the ground. Extreme examples are a basin and a cliff, where water infiltrates much quicker into a basin than a cliff that has the same soil properties. Because saturated soil does not have the capacity to take more water, runoff is generally greater over saturated soil. Clay-rich soil cannot accept infiltration as quickly as gravel-rich soil.
11.5.3 Drainage Patterns
The pattern of tributaries within a region is called drainage pattern. They depend largely on the type of rock beneath, and on structures within that rock (such as folds and faults). The main types of drainage patterns are dendritic, trellis, rectangular, radial, and deranged. Dendritic patterns are the most common and develop in areas where the underlying rock or sediments are uniform in character, mostly flat lying, and can be eroded equally easily in all directions. Examples are alluvial sediments or flat lying sedimentary rocks. Trellis patterns typically develop where sedimentary rocks have been folded or tilted and then eroded to varying degrees depending on their strength. The Appalachian Mountains in eastern United States have many good examples of trellis drainage. Rectangular patterns develop in areas that have very little topography and a system of bedding planes, joints, or faults that form a rectangular network. A radial pattern forms when streams flow away from a central high point such as a mountain top or volcano, with the individual streams typically having dendritic drainage patterns. In places with extensive limestone deposits, streams can disappear into the groundwater via caves and subterranean drainage and this creates a deranged pattern [4].

11.5.4 Fluvial Processes
Fluvial processes dictate how a stream behaves and include factors controlling fluvial sediment production, transport, and deposition. Fluvial processes include velocity, slope and gradient, erosion, transportation, deposition, stream equilibrium, and base level.
Streams can be divided into three main zones: the many smaller tributaries in the source area, the main trunk stream in the floodplain and the distributaries at the mouth of the stream. Major stream systems like the Mississippi are composed of many source areas, many tributaries and trunk streams, all coalescing into the one main stream draining the region. The zones of a stream are defined as 1) the zone of sediment production (erosion), 2) the zone of transport, and 3) the zone of deposition. The zone of sediment production is located in the headwaters of the stream. In the zone of sediment transport, there is a general balance between erosion of the finer sediment in its channel and transport of sediment across the floodplain. Streams eventually flow into the ocean or end in quiet water with a delta which is a zone of sediment deposition located at the mouth of a stream [6]. The longitudinal profile of a stream is a plot of the elevation of the stream channel at all points along its course and illustrates the location of the three zones [22]
Zone of Sediment Production
The zone of sediment production is located in the headwaters of a stream where rills and gullies erode sediment and contribute to larger tributary streams. These tributaries carry sediment and water further downstream to the main trunk of the stream. Tributaries at the headwaters have the steepest gradient; erosion there produces considerable sediment carried b the stream. Headwater streams tend to be narrow and straight with small or non-existent floodplains adjacent to the channel. Since the zone of sediment production is generally the steepest part of the stream, headwaters are generally located in relatively high elevations. The Rocky Mountains of Wyoming and Colorado west of the Continental Divide contain much of the headwaters for the Colorado River which then flows from Colorado through Utah and Arizona to Mexico. Headwaters of the Mississippi river system lie east of the Continental Divide in the Rocky Mountains and west of the Appalachian Divide.
Zone of Sediment TransPORT
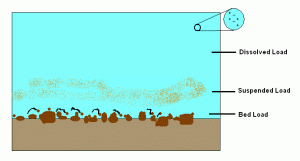
Streams transport sediment great distances from the headwaters to the ocean, the ultimate depositional basins. Sediment transportation is directly related to stream gradient and velocity. Faster and steeper streams can transport larger sediment grains. When velocity slows down, larger sediments settle to the channel bottom. When the velocity increases, those larger sediments are entrained and move again.
Transported sediments are grouped into bedload, suspended load, and dissolved load as illustrated in the above image. Sediments moved along the channel bottom are the bedload that typically consists of the largest and densest particles. Bedload is moved by saltation (bouncing) and traction (being pushed or rolled along by the force of the flow). Smaller particles are picked up by flowing water and carried in suspension as suspended load. The particle size that is carried in suspended and bedload depends on the flow velocity of the stream. Dissolved load in a stream is the total of the ions in solution from chemical weathering, including such common ions such as bicarbonate (-HCO3-), calcium (Ca+2), chloride (Cl-1), potassium (K+1), and sodium (Na+1). The amounts of these ions are not affected by flow velocity.
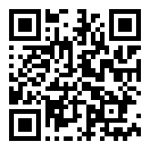

A floodplain is the flat area of land adjacent to a stream channel inundated with flood water on a regular basis. Stream flooding is a natural process that adds sediment to floodplains. A stream typically reaches its greatest velocity when it is close to flooding, known as the bankfull stage. As soon as the flooding stream overtops its banks and flows onto its floodplain, the velocity decreases. Sediment that was being carried by the swiftly moving water is deposited at the edge of the channel, forming a low ridge or natural levée. In addition, sediments are added to the floodplain during this flooding process contributing to fertile soils [4].
Zone of SEDIMENT Deposition
Deposition occurs when bedload and suspended load come to rest on the bottom of the stream channel, lake, or ocean due to decrease in stream gradient and reduction in velocity. While both deposition and erosion occur in the zone of transport such as on point bars and cut banks, ultimate deposition where the stream reaches a lake or ocean. Landforms called deltas form where the stream enters quiet water composed of the finest sediment such as fine sand, silt, and clay.
Equilibrium and Base Level
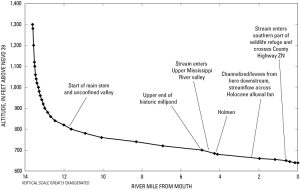
All three stream zones are present in the typical longitudinal profile of a stream which plots the elevation of the channel at all points along its course (see figure). All streams have a long profile. The long profile shows the stream gradient from headwater to mouth. All streams attempt to achieve an energetic balance among erosion, transport, gradient, velocity, discharge, and channel characteristics along the stream’s profile. This balance is called equilibrium, a state called grade.
Another factor influencing equilibrium is base level, the elevation of the stream's mouth representing the lowest level to which a stream can erode. The ultimate base level is, of course, sea-level. A lake or reservoir may also represent base level for a stream entering it. The Great Basin of western Utah, Nevada, and parts of some surrounding states contains no outlets to the sea and provides internal base levels for streams within it. Base level for a stream entering the ocean changes if sea-level rises or falls. Base level also changes if a natural or human-made dam is added along a stream's profile. When base level is lowered, a stream will cut down and deepen its channel. When base level rises, deposition increases as the stream adjusts attempting to establish a new state of equilibrium. A stream that has approximately achieved equilibrium is called a graded stream.
11.5.5 Fluvial Landforms
Stream landforms are the land features formed on the surface by either erosion or deposition. The stream-related landforms described here are primarily related to channel types.
Channel Types
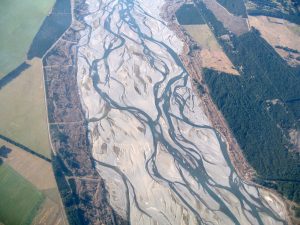
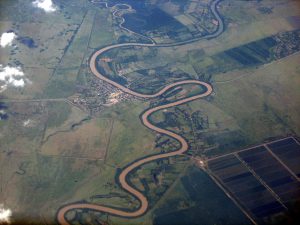
Stream channels can be straight, braided, meandering, or entrenched. The gradient, sediment load, discharge, and location of base level all influence channel type. Straight channels are relatively straight, located near the headwaters, have steep gradients, low discharge, and narrow V-shaped valleys. Examples of these are located in mountainous areas.
Braided streams have multiple channels splitting and recombining around numerous mid-channel bars. These are found in floodplains with low gradients in areas with near sources of coarse sediment such as trunk streams draining mountains or in front of glaciers.
Meandering streams have a single channel that curves back and forth like a snake within its floodplain where it emerges from its headwaters into the zone of transport. Meandering streams are dynamic creating a wide floodplain by eroding and extending meander loops side-to-side. The highest velocity water is located on the outside of a meander bend. Erosion of the outside of the curve creates a feature called a cut bank and the meander extends its loop wider by this erosion.
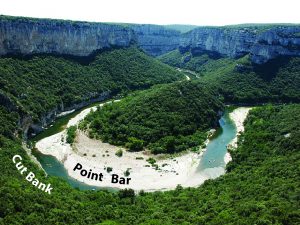
The thalweg of the stream is the deepest part of the stream channel. In the straight parts of the channel, the thalweg and highest velocity are in the center of the channel. But at the bend of a meandering stream, the thalweg shifts toward the cut bank. Opposite the cutbank on the inside bend of the channel is the lowest stream velocity and is an area of deposition called a point bar.
In areas of tectonic uplift such as on the Colorado Plateau, meandering streams that once flowed on the plateau surface have become entrenched or incised as uplift occurred and the stream cut its meandering channel down into bedrock. Over the past several million years, the Colorado River and its tributaries have incised into the flat lying rocks of the plateau by hundreds, even thousands of feet creating deep canyons including the Grand Canyon in Arizona.
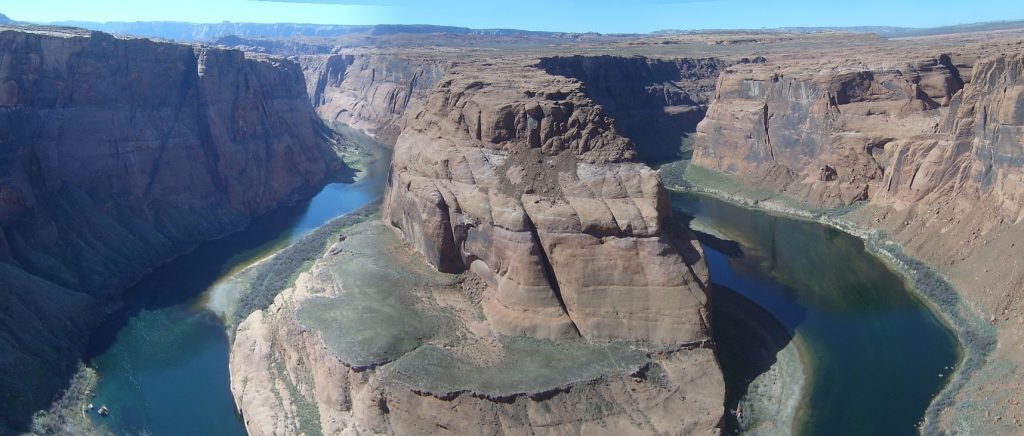
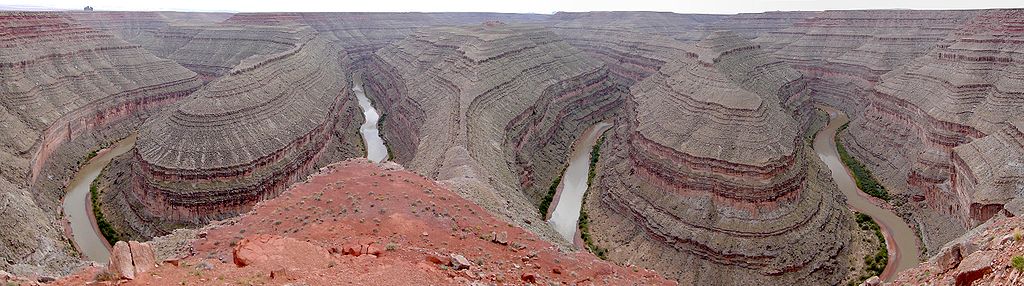
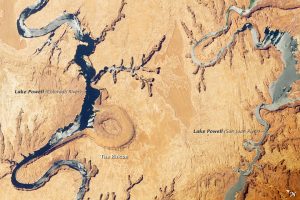
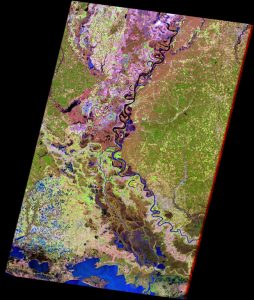
Many fluvial landforms occur on a floodplain associated with a meandering stream. Meander activity and regular flooding contribute to widening the floodplain by eroding adjacent uplands. The stream channels are confined by natural levees that have been built up over many years of regular flooding. Natural levees can isolate and direct flow from tributary channels on the floodplain from immediately reaching the main channel. These isolated streams are called yazoo streams and flow parallel to the main trunk stream until there is an opening in the levee to allow for a belated confluence.
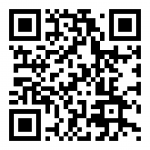
To limit flooding, humans build artificial levees on flood plains. Sediment that breaches the levees during flood stage is called crevasse splays and delivers silt and clay onto the floodplain. These deposits are rich in nutrients and often make good farm land. When floodwaters crest over human-made levees, the levees quickly erode with potentially catastrophic impacts. Because of the good soils, farmers regularly return after floods and rebuild year after year.
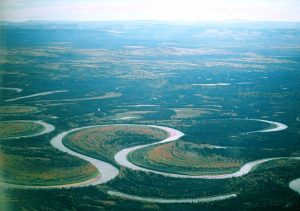
Through erosion on the outsides of the meanders and deposition on the insides, the channels of meandering streams move back and forth across their floodplain over time. On very broad floodplains with very low gradients, the meander bends can become so extreme that they cut across themselves at a narrow neck (see figure) called a cutoff. The former channel becomes isolated and forms an oxbow lake seen on the right of the figure. Eventually the oxbow lake fills in with sediment and becomes a wetland and eventually a meander scar. Stream meanders can migrate and form oxbow lakes in a relatively short amount of time. Where stream channels form geographic and political boundaries, this shifting of channels can cause conflicts.
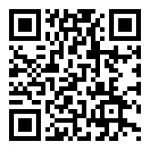
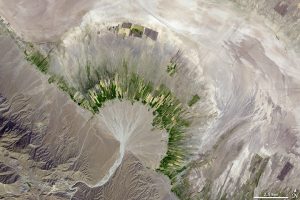
Alluvial fans are a depositional landform created where streams emerge from mountain canyons into a valley. The channel that had been confined by the canyon walls is no longer confined, slows down and spreads out, dropping its bedload of all sizes, forming a delta in the air of the valley. As distributary channels fill with sediment, the stream is diverted laterally, and the alluvial fan develops into a cone shaped landform with distributaries radiating from the canyon mouth. Alluvial fans are common in the dry climates of the West where ephemeral streams emerge from canyons in the ranges of the Basin and Range.
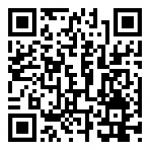
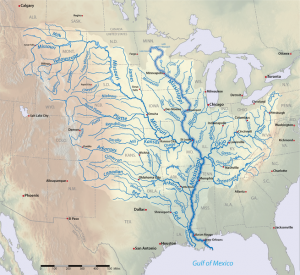
A delta is formedwhen a stream reaches a quieter body of water such as a lake or the ocean and the bedload and suspended load is deposited. If wave erosion from the water body is greater than deposition from the river, a delta will not form. The largest and most famous delta in the United States is the Mississippi River delta formed where the Mississippi River flows into the Gulf of Mexico. The Mississippi River drainage basin is the largest in North America, draining 41% of the contiguous United States [24]. Because of the large drainage area, the river carries a large amount of sediment. The Mississippi River is a major shipping route and human engineering has ensured that the channel has been artificially straightened and remains fixed within the floodplain. The river is now 229 km shorter than it was before humans began engineering it [24]. Because of these restraints, the delta is now focused on one trunk channel and has created a “bird’s foot” pattern. The two NASA images below of the delta show how the shoreline has retreated and land was inundated with water while deposition of sediment was focused at end of the distributaries. These images have changed over a 25 year period from 1976 to 2001. These are stark changes illustrating sea-level rise and land subsidence from the compaction of peat due to the lack of sediment resupply [25].
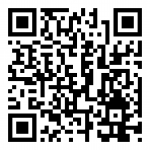
The formation of the Mississippi River delta started about 7500 years ago when postglacial sea level stopped rising. In the past 7000 years, prior to anthropogenic modifications, the Mississippi River delta formed several sequential lobes. The river abandoned each lobe for a more preferred route to the Gulf of Mexico. These delta lobes were reworked by the ocean waves of the Gulf of Mexico [26]. After each lobe was abandoned by the river, isostatic depression and compaction of the sediments caused basin subsidence and the land to sink.
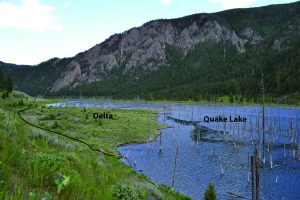
A clear example of how deltas form came from an earthquake. During the 1959 Madison Canyon 7.5 magnitude earthquake in Montana, a large landslide dammed the Madison River forming Quake Lake still there today [27]. A small tributary stream that once flowed into the Madison River, now flows into Quake Lake forming a delta composed of coarse sediment actively eroded from the mountainous upthrown block to the north.
Deltas can be further categorized as wave-dominated or tide-dominated. Wave-dominated deltas occur where the tides are small and wave energy dominates. An example is the Nile River delta in the Mediterranean Sea that has the classic shape of the Greek character (Δ) from which the landform is named. A tide-dominated delta forms when ocean tides are powerful and influence the shape of the delta. For example, Ganges-Brahmaputra Delta in the Bay of Bengal (near India and Bangladesh) is the world’s largest delta and mangrove swamp called the Sundarban [29].
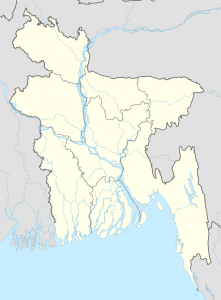
At the Sundarban Delta in Bangladesh, tidal forces create linear intrusions of seawater into the delta. This delta also holds the world’s largest mangrove swamp.
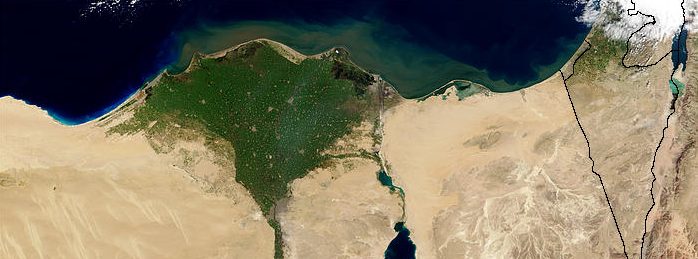
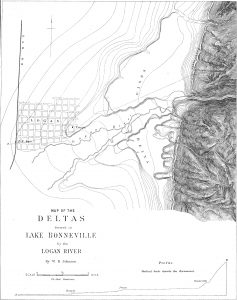
Lake Bonneville was a large, pluvial lake that occupied the western half of Utah and parts of eastern Nevada from about 30,000 to 12,000 years ago. The lake filled to a maximum elevation as great as approximately 5100 feet above mean sea level, filling the basins, leaving the mountains exposed, many as islands. The presence of the lake allowed for deposition of both fine grained lake mud and silt and coarse gravels from the mountains. Variations in lake level were controlled by regional climate and a catastrophic failure of Lake Bonneville’s main outlet, Red Rock Pass [31]. during extended periods of time in which the lake level remained stable, wave-cut terraces were produced that can be seen today on the flanks of many mountains in the region. Significant deltas formed at the mouths of major canyons in Salt Lake, Cache, and other Utah valleys. The Great Salt Lake is the remnant of Lake Bonneville and cities have built up on these delta deposits.
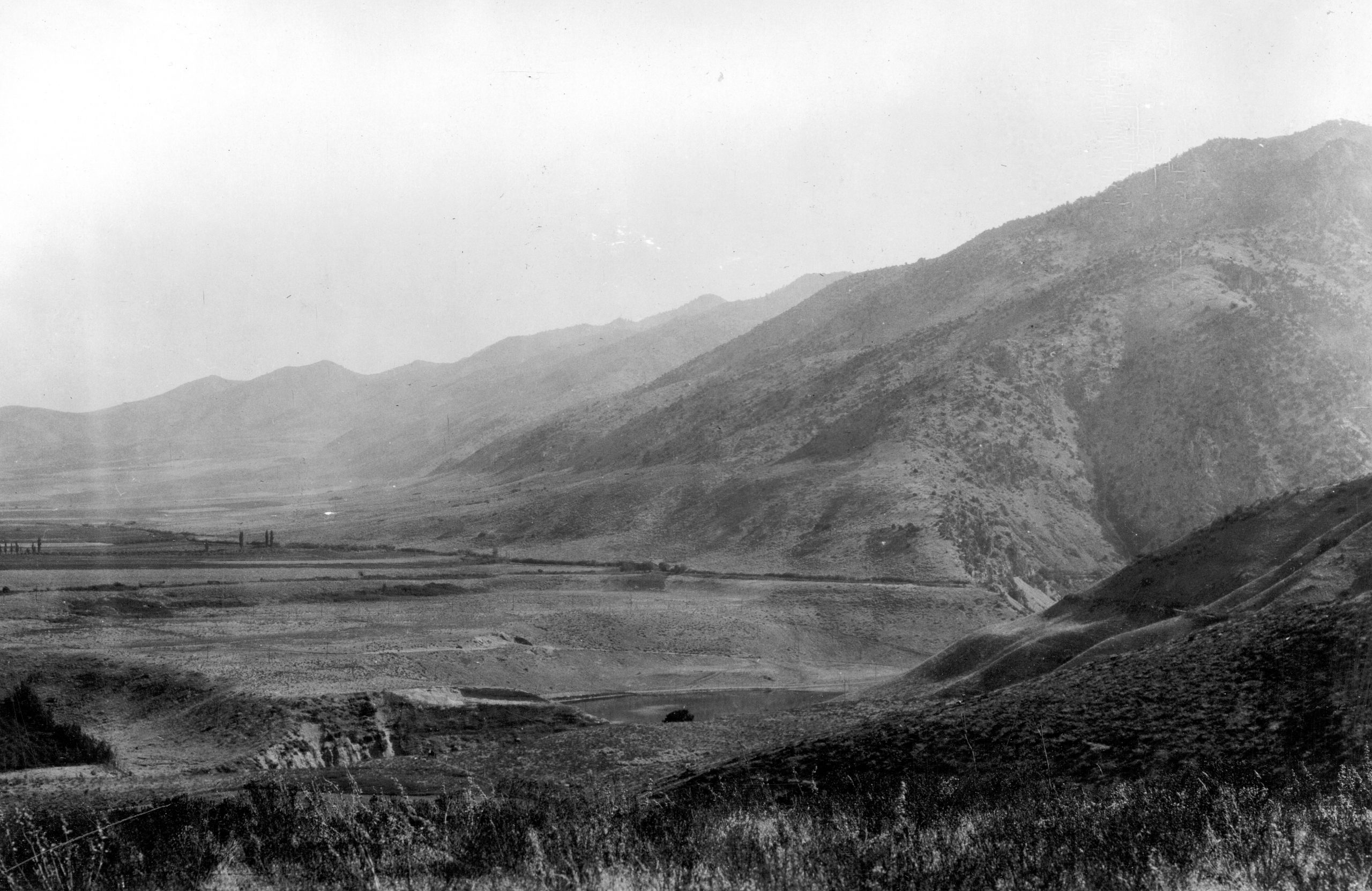
Stream terraces are remnants of older floodplains located above the existing floodplain and river. Like entrenched meanders, stream terraces form when uplift occurs or base level drops and streams erode downward, their meanders widening a new flood plain. Stream terraces can also form from extreme flood events associated with retreating glaciers. A classic example of multiple stream terraces are along the Snake River in Grand Teton National Park in Wyoming [32; 33].
![]() |
![]() |
Take this quiz to check your comprehension of this section.
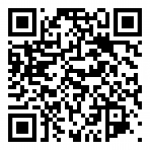
11.6 Groundwater
Groundwater is an important source of freshwater. It can be found at varying depths in all places under the ground, but is limited by extractable quantity and quality.
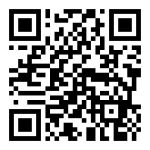
11.6.1 Porosity and Permeability
An aquifer is a rock unit that contains extractable ground water. A good aquifer must be both porous and permeable. Porosity is the space between grains that can hold water, expressed as the percentage of open space in the total volume of the rock. Permeability comes from connectivity of the spaces that allows water to move in the aquifer. Porosity can occur as primary porosity, as space between sand grains or vesicles in volcanic rocks, or secondary porosity as fractures or dissolved spaces in rock). Compaction and cementation during lithification of sediments reduces porosity (see chapter 5.3).
A combination of a place to contain water (porosity) and the ability to move water (permeability) makes a good aquifer—a rock unit or sediment that allows extraction of groundwater. Well-sorted sediments have higher porosity because there are not smaller sediment particles filling in the spaces between the larger particles. Shales made of clays generally have high porosity, but the pores are poorly connected, thereby causing low permeability.
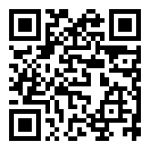
While permeability is an important measure of a porous material’s ability to transmit water, hydraulic conductivity is more commonly used by geologists to measure how easily a fluid is transmitted. Hydraulic conductivity measures both the permeability of the porous material and the properties of the water, or whatever fluid is being transmitted like oil or gas. Because hydraulic conductivity also measures the properties of the fluid, such as viscosity, it is used by both petroleum geologists and hydrogeologists to describe both the production capability of oil reservoirs and of aquifers. High hydraulic conductivity indicates that fluid transmits rapidly through an aquifer.
11.6.2 Aquifers
Aquifers are rock layers with sufficient porosity and permeability to allow water to be both contained and move within them. For rock or sediment to be considered an aquifer, its pores must be at least partially filled with water and it must be permeable enough to transmit water. Drinking water aquifers must also contain potable water. Aquifers can vary dramatically in scale, from spanning several formations covering large regions to being a local formation in a limited area. Aquifers adequate for water supply are both permeable, porous, and potable.
11.6.3 Groundwater Flow
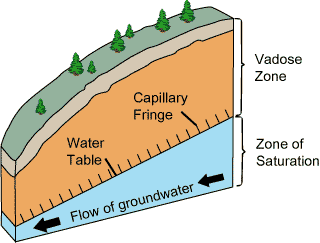
When surface water infiltrates or seeps into the ground, it usually enters the unsaturated zone also called the vadose zone, or zone of aeration. The vadose zone is the volume of geologic material between the land surface and the zone of saturation where the pore spaces are not completely filled with water [34]. Plant roots inhabit the upper vadose zone and fluid pressure in the pores is less than atmospheric pressure. Below the vadose zone is the capillary fringe. Capillary fringe is the usually thin zone below the vadose zone where the pores are completely filled with water (saturation), but the fluid pressure is less than atmospheric pressure. The pores in the capillary fringe are filled because of capillary action, which occurs because of a combination of adhesion and cohesion. Below the capillary fringe is the saturated zone or phreatic zone, where the pores are completely saturated and the fluid in the pores is at or above atmospheric pressure. The interface between the capillary fringe and the saturated zone marks the location of the water table.
Wells are conduits that extend into the ground with openings to the aquifers, to extract from, measure, and sometimes add water to the aquifer. Wells are generally the way that geologists and hydrologist measure the depth to groundwater from the land surface as well as withdraw water from aquifers.
Water is found throughout the pore spaces in sediments and bedrock. The water table is the area below which the pores are fully saturated with water. The simplest case of a water table is when the aquifer is unconfined, meaning it does not have a confining layer above it. Confining layers can pressurize aquifers by trapping water that is recharged at a higher elevation underneath the confining layer, allowing for a potentiometric surface higher than the top of the aquifer, and sometimes higher than the land surface.
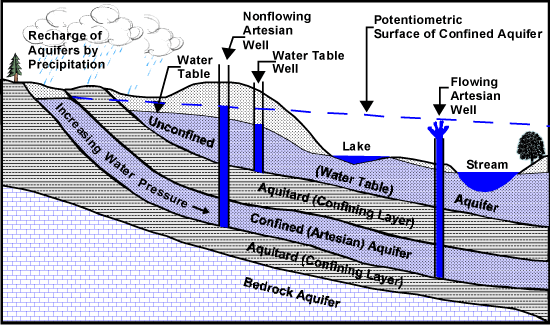
A confining layer is a low permeability layer above and/or below an aquifer that restricts the water from moving in and out of the aquifer. Confining layers include aquicludes, which are so impermeable that no water travels through them, and aquitards, which significantly decrease the speed at which water travels through them. The potentiometric surface represents the height that water would rise in a well penetrating the pressurized aquifer system. Breaches in the pressurized aquifer system, like faults or wells, can cause springs or flowing wells, also known as artesian wells.
The water table will generally mirror surface topography, though more subdued, because hydrostatic pressure is equal to atmospheric pressure along the surface of the water table. If the water table intersects the ground surface the result will be water at the surface in the form of a gaining stream, spring, lake, or wetland. The water table intersects the channel for gaining streams which then gains water from the water table. The channels for losing streams lie below the water table, thus losing streams lose water to the water table. Losing streams may be seasonal during a dry season or ephemeral in dry climates where they may normally be dry and carry water only after rain storms. Ephemeral streams pose a serious danger of flash flooding in dry climates.
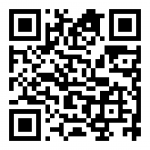
Mentioned in the video is the USGS Groundwater Watch site.
Using wells, geologists measure the water table’s height and the potentiometric surface. Graphs of the depth to groundwater over time, are known as hydrographs and show changes in the water table over time. Well-water level is controlled by many factors and can change very frequently, even every minute, seasonally, and over longer periods of time.
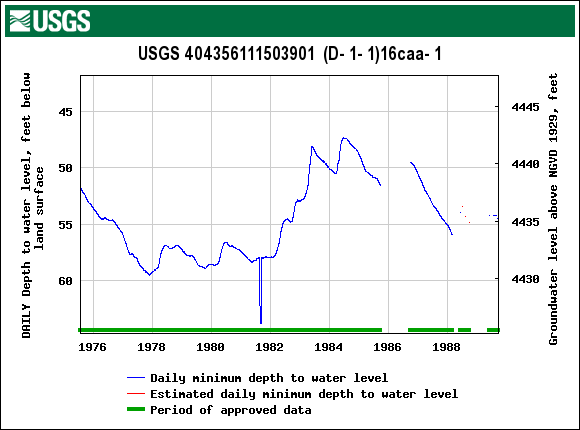
In 1856, French engineer Henry Darcy developed a hypothesis to show how discharge through a porous medium is controlled by permeability, pressure, and cross- sectional area. To prove this relationship, Darcy experimented with tubes of packed sediment with water running through them. The results of his experiments empirically established a quantitative measure of hydraulic conductivity and discharge that is known as Darcy’s law. The relationships described by Darcy’s Law have close similarities to Fourier's law in the field of heat conduction, Ohm's law in the field of electrical networks, or Fick's law in diffusion theory.
Q=KA(Δh/L)
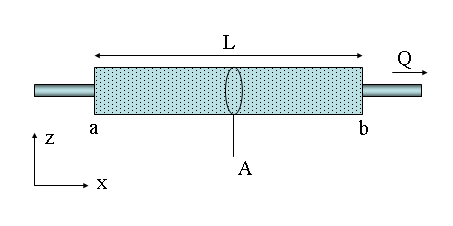
- Q = flow (volume/time)
- K = hydraulic conductivity (length/time)
- A = cross-sectional area of flow (area)
- Δh = change in pressure head (pressure difference)
- L = distance between pressure (h) measurements (length)
- Δh/L is commonly referred to as the hydraulic gradient
Pumping water from an unconfined aquifer lowers the water table. Pumping water from a confined aquifer lowers the pressure and/or potentiometric surface around the well. In an unconfined aquifer, the water table is lowered as water is removed from the aquifer near the well producing drawdown and a cone of depression (see figure). In a confined aquifer, pumping on an artesian well reduces the pressure or potentiometric surface around the well.
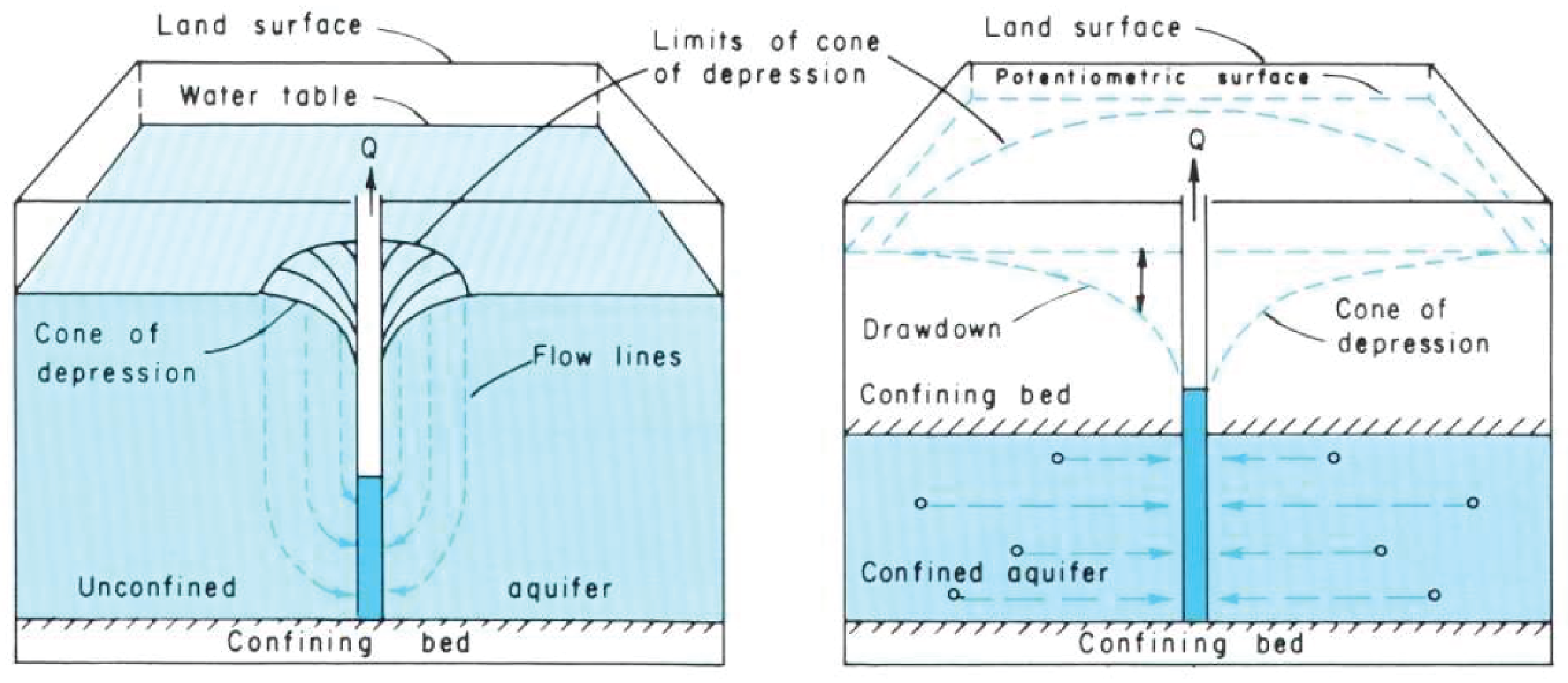
When one cone of depression intersects another cone of depression or a barrier feature like an impermeable mountain block, drawdown is intensified. When a cone of depression intersects a recharge zone, the cone of depression is lessened.
11.6.4 Recharge
The recharge area is where surface water enters an aquifer through the process of infiltration. Recharge areas are generally topographically high locations of an aquifer. They are characterized by losing streams and permeable rock that allows infiltration into the aquifer. Recharge areas mark the beginning of groundwater flow paths.
In the Basin and Range Province, recharge areas for the unconsolidated aquifers of the valleys are along mountain foothills. In the foothills of Salt Lake Valley, losing streams contribute water to the gravel-rich deltaic deposits of ancient Lake Bonneville, in some cases feeding artesian wells in the Salt Lake Valley.
An aquifer management practice is to induce recharge through storage and recovery. Geologists and hydrologists can increase the recharge rate into an aquifer system using injection wells and infiltration galleries or basins [35]. Injection wells pump water into an aquifer where it can be stored. Injection wells are regulated by state and federal governments to ensure that the injected water is not negatively impacting the quality or supply of the existing groundwater in the aquifer. Some aquifers can store significant quantities of water, allowing water managers to use the aquifer system like a surface reservoir. Water is stored in the aquifer during periods of low water demand and high water supply and later extracted during times of high water demand and low water supply.
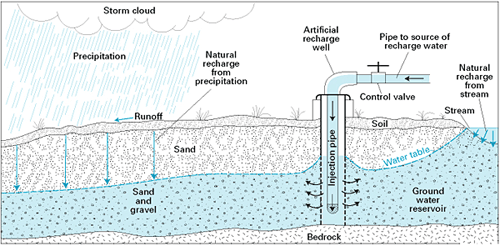
11.6.5 Discharge
Discharge areas are where the water table or potentiometric surface intersects the land surface. Discharge areas mark the end of groundwater flow paths. These areas are characterized by springs, flowing (artesian) wells, gaining streams, and playas in the dry valley basins of the Basin and Range Province of the western United States.
11.6.6 Groundwater mining and subsidence
Like other natural resources on our planet, the quantity of fresh and potable water is finite. The only natural source of water on land is from the sky in the form of precipitation. In many places, groundwater is being extracted faster than it is being replenished. When groundwater is extracted faster than it is recharged, groundwater levels and potentiometric surfaces decline, and discharge areas diminish or dry up completely. Regional pumping-induced groundwater decline is known as groundwater mining or groundwater overdraft. Groundwater mining is a serious situation and can lead to dry wells, reduced spring and stream flow, and subsidence. Groundwater mining is happening is places where more water is extracted by pumping than is being replenished by precipitation, and the water table is continually lowered. In these situations, groundwater must be viewed as a ore body and in its depletion, the possibility of producing ghost towns.
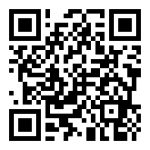
In many places, water actually helps hold up an aquifer’s skeleton by the water pressure exerted on the grains in an aquifer. This pressure is called pore pressure and comes from the weight of overlying water. If pore pressure decreases because of groundwater mining, the aquifer can compact, causing the surface of the ground to sink. Areas especially susceptible to this effect are aquifers made of unconsolidated sediments. Unconsolidated sediments with multiple layers of clay and other fine-grained material are at higher risk because when water is drained, clay compacts considerably [36; 37].
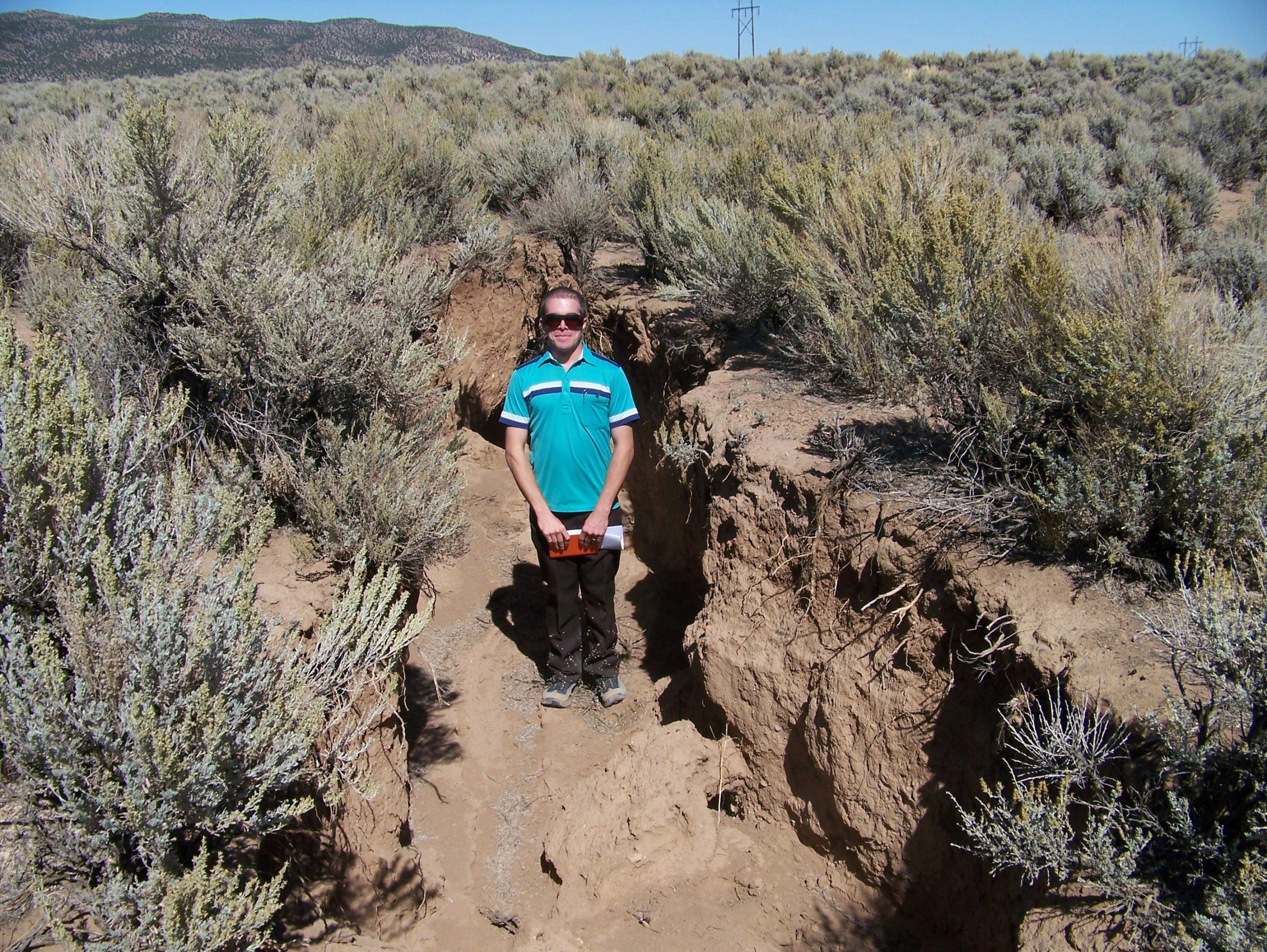
Subsidence from groundwater mining has been documented in southwestern Utah, notably Cedar Valley, Iron County, Utah. Groundwater levels have declined more than 100 feet in certain parts of Cedar Valley, causing earth fissures and measurable amounts of land subsidence.
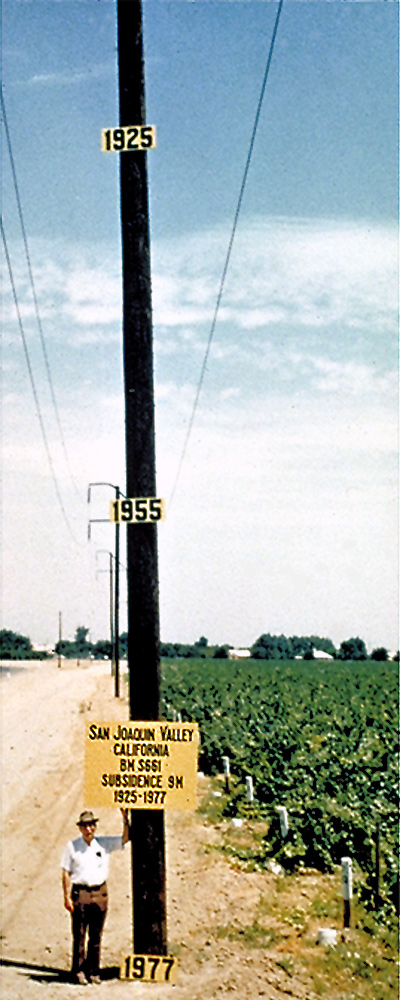
This photo shows documentation of subsidence from pumping of groundwater for irrigation in the Central Valley in California. The pole shows subsidence from groundwater pumping over a period of time.
Take this quiz to check your comprehension of this section.
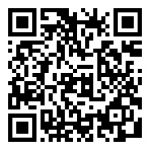
11.7 Water Contamination and Remediation
Water can be contaminated by natural features like mineral-rich geologic formations and by human activities such as agriculture, industrial operations, landfills, animal operations, and sewage treatment processes, among many other things. As water runs over the land or infiltrates into the ground, it dissolves material left behind by these potential contaminant sources. There are three major groups of contamination: organic and inorganic chemicals and biological agents. Small sediments that cloud water, causing turbidity, is also an issue with some wells, but it is not considered contamination. The risks and type of remediation for a contaminant depends on the type of chemicals present.
Contamination occurs as point-source and nonpoint-source pollution. Point source pollution can be attributed to a single, definable source, while nonpoint source pollution is from multiple dispersed sources. Point sources include waste disposal sites, storage tanks, sewage treatment plants, and chemical spills. Nonpoint sources are dispersed and indiscreet, where the whole of the contribution of pollutants is harmful, but the individual components do not have harmful concentrations of pollutants. A good example of nonpoint pollution is residential areas, where lawn fertilizer on one person’s yard may not contribute much pollution to the system, but the combined effect of many residents using fertilizer can lead to significant nonpoint pollution. Other nonpoint sources include nutrients (nitrate and phosphate), herbicides, pesticides contributed by farming, nitrate contributed by animal operations, and nitrate contributed by septic systems.
Organic chemicals are common pollutants. They consist of strands and rings of carbon atoms, usually connected by covalent bonds. Other types of atoms, like chlorine, and molecules, like hydroxide (OH-), are attached to the strands and rings. The number and arrangement of atoms will decide how the chemical behaves in the environment, its danger to humans or ecosystems, and where the chemical ends up in the environment. The different arrangements of carbon allow for tens of thousands of organic chemicals, many of which have never been studied for negative effects on human health or the environment. Common organic pollutants are herbicides and pesticides, pharmaceuticals, fuel, and industrial solvents and cleansers.
Organic chemicals include surfactants such as cleaning agents and synthetic hormones associated with pharmaceuticals, which can act as endocrine disruptors. Endocrine disruptors mimic hormones, and can cause long-term effects in developing sexual reproduction systems in developing animals. Only very small quantities of endocrine disruptors are needed to cause significant changes in animal populations.
An example of organic chemical contamination is the Love Canal, Niagara Falls, New York. From 1942 to 1952, the Hooker Chemical Company disposed of over 21,337 mt (21,000 t) of chemical waste, including chlorinated hydrocarbons, into a canal and covered it with a thin layer of clay. Chlorinated hydrocarbons are a large group of organic chemicals that have chlorine functional groups, most of which are toxic and carcinogenic to humans. The company sold the land to the New York School Board, who developed it into a neighborhood. After residents began to suffer from serious health ailments and pools of oily fluid started rising into residents’ basements, the neighborhood had to be evacuated. This site became a U.S. Environmental Protection Agency Superfund site, a site with federal funding and oversight to ensure its cleanup.
Inorganic chemicals are another set of chemical pollutants. They can contain carbon atoms, but not in long strands or links. Inorganic contaminants include chloride, arsenic, and nitrate (NO3). Nutrients can be from geologic material, like phosphorous-rich rock, but are most often sourced from fertilizer and animal and human waste. Untreated sewage and agricultural runoff contain concentrates of nitrogen and phosphorus which are essential for the growth of microorganisms. Nutrients like nitrate and phosphate in surface water can promote growth of microbes, like blue-green algae (cyanobacteria), which in turn use oxygen and create toxins (microcystins and anatoxins) in lakes [38]. This process is known as eutrophication.
Metals are common inorganic contaminants. Lead, mercury, and arsenic are some of the more problematic inorganic groundwater contaminants. Bangladesh has a well documented case of arsenic contamination from natural geologic material dissolving into the groundwater. Acid-mine drainage can also cause significant inorganic contamination (see Chapter 16).
Salt, typically sodium chloride, is a common inorganic contaminant. It can be introduced into groundwater from natural sources, such as evaporite deposits like the Arapien Shale of Utah, or from anthropogenic sources like the salts applied to roads in the winter to keep ice from forming. Salt contamination can also occur near ocean coasts from saltwater intruding into the cones of depression around fresh groundwater pumping, inducing the encroachment of saltwater into the freshwater body.
Biological agents are another common groundwater contaminant which includes harmful bacteria and viruses. A common bacteria contaminant is Escherichia coli (E. coli). Generally, harmful bacteria are not present in groundwater unless the groundwater source is closely connected with a contaminated surface source, such as a septic system. Karst, landforms created from dissolved limestone, is especially susceptible to this form of contamination, because water moves relatively quickly through the conduits of dissolved limestone. Bacteria can also be used for remediation.
Table. Groundwater contaminants.
Remediation is the act of cleaning contamination. Hydrologists use three types of remediation: biological, chemical, and physical. Biological remediation uses specific strains of bacteria to break down a contaminant into safer chemicals. This type of remediation is usually used on organic chemicals, but also works on reducing or oxidizing inorganic chemicals like nitrate. Phytoremediation is a type of bioremediation that uses plants to absorb the chemicals over time.
Chemical remediation uses chemicals to remove the contaminant or make it less harmful. One example is to use a reactive barrier, a permeable wall in the ground or at a discharge point that chemically reacts with contaminants in the water. Reactive barriers made of limestone can increase the pH of acid mine drainage, making the water less acidic and more basic, which removes dissolved contaminants by precipitation into solid form.
Physical remediation consists of removing the contaminated water and either treating it with filtration, called pump-and-treat, or disposing of it. All of these options are technically complex, expensive, and difficult, with physical remediation typically being the most costly.
Take this quiz to check your comprehension of this section.
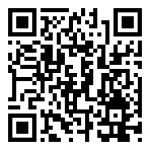
11.8 Karst
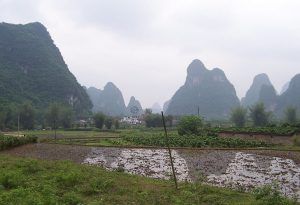
Karst refers to landscapes and hydrologic features created by the dissolving of limestone. Karst can be found anywhere there is limestone and other soluble subterranean substances like salt deposits. Dissolving of limestone creates features like sinkholes, caverns, disappearing streams, and towers.
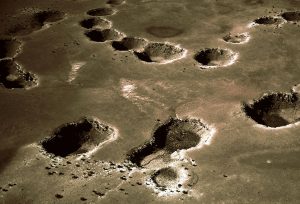
Dissolving of underlying salt deposits has caused sinkholes to form in the Kaibab Limestone on the Colorado Plateau in Arizona.
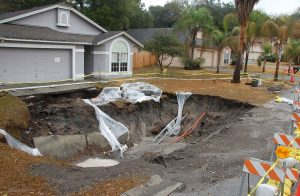
Collapse of the surface into an underground cavern caused this sinkhole in the front yard of a home in Florida.
CO2 in the atmosphere dissolves readily in the water droplets that form clouds from which precipitation comes in the form of rain and snow. This precipitation is slightly acidic with carbonic acid. Karst forms when carbonic acid dissolves calcite (calcium carbonate) in limestone.
H2O + CO2 = H2CO3
Water + Carbon Dioxide Gas equals Carbonic Acid in Water
CaCO3 + H2CO3 = Ca2++ 2HCO3 -1
Solid Calcite + Carbonic Acid in Water Dissolved equals Calcium Ion + Dissolved Bicarbonate Ion
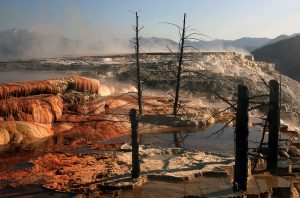
After the slightly acidic water dissolves the calcite, changes in temperature or gas content in the water can cause the water to redeposit the calcite in a different place as tufa (travertine), often deposited by a spring or in a cave. Speleothems are secondary deposits, typically made of travertine, deposited in a cave. Travertine speleothems form by water dripping through cracks and dissolved openings in caves and evaporating, leaving behind the travertine deposits. Speleothems commonly occur in the form of stalactites, when extending from the ceiling, and stalagmites, when standing up from the floor.
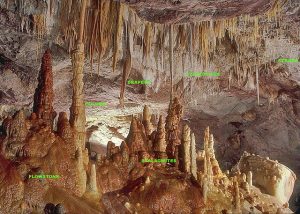
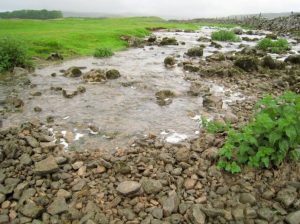
Surface water enters the karst system through sinkholes, losing streams, and disappearing streams. Changes in base level can cause rivers running over limestone to dissolve the limestone and sink into the ground. As the water continues to dissolve its way through the limestone, it can leave behind intricate networks of caves and narrow passages. Often dissolution will follow and expand fractures in the limestone. Water exits the karst system as springs and rises. In mountainous terrane, dissolution can extend all the way through the vertical profile of the mountain, with caverns dropping thousands of feet.
Take this quiz to check your comprehension of this section.
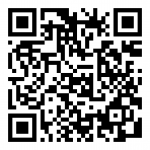
Summary
Water is essential for all living things. It continuously cycles through the atmosphere, over land, and through the ground. In much of the United States and other countries, water is managed through a system of regional laws and regulations and distributed on paper in a system collectively known as “water rights”. Surface water follows a watershed, which is separate from other areas by its divides (highest ridges). Groundwater exists in the pores within rocks and sediment. It moves predominantly due to pressure and gravitational gradients through the rock. Human and natural causes can make water unsuitable for consumption. There are different ways to deal with this contamination. Karst is when limestone is dissolved by water, forming caves and sinkholes.
Take this quiz to check your comprehension of this Chapter.
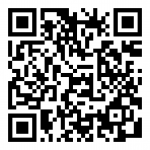
References
- Brush, L.M., Jr, 1961, Drainage basins, channels, and flow characteristics of selected streams in central Pennsylvania: pubs.er.usgs.gov.
- Charlton, R., 2007, Fundamentals of fluvial geomorphology: Taylor & Francis.
- Cirrus Ecological Solutions, 2009, Jordan River TMDL: Utah State Division of Water Quality.
- Earle, S., 2015, Physical geology OER textbook: BC Campus OpenEd.
- EPA, 2009, Water on Tap-What You Need to Know: U.S. Environmental Protection Agency.
- Fagan, B., 2012, Elixir: A history of water and humankind: Bloomsbury Press.
- Fairbridge, R.W., 1968, Yazoo rivers or streams, in Geomorphology: Springer Berlin Heidelberg Encyclopedia of Earth Science, p. 1238–1239.
- Freeze, A.R., and Cherry, J.A., 1979, Groundwater: Prentice Hall.
- Galloway, D., Jones, D.R., and Ingebritsen, S.E., 1999, Land subsidence in the United States: U.S. Geological Survey Circular 1182.
- Galloway, W.E., Whiteaker, T.L., and Ganey-Curry, P., 2011, History of Cenozoic North American drainage basin evolution, sediment yield, and accumulation in the Gulf of Mexico basin: Geosphere, v. 7, no. 4, p. 938–973.
- Gilbert, G.K., 1890, Lake Bonneville: United States Geological Survey, 438 p.
- Gleick, P.H., 1993, Water in Crisis: A Guide to the World’s Fresh Water Resources: Oxford University Press.
- Hadley, G., 1735, Concerning the cause of the general trade-winds: By Geo. Hadley, Esq; FRS: Philosophical Transactions, v. 39, no. 436–444, p. 58–62.
- Halvorson, S.F., and James Steenburgh, W., 1999, Climatology of lake-effect snowstorms of the Great Salt Lake: University of Utah.
- Heath, R.C., 1983, Basic ground-water hydrology: U.S. Geological Survey Water-Supply Paper 2220, 91 p.
- Hobbs, W.H., and Fisk, H.N., 1947, Geological Investigation of the Alluvial Valley of the Lower Mississippi River: JSTOR.
- Knudsen, T., Inkenbrandt, P., Lund, W., Lowe, M., and Bowman, S., 2014, Investigation of land subsidence and earth fissures in Cedar Valley, Iron County, Utah: Utah Geological Survey Special Study 150.
- Lorenz, E.N., 1955, Available potential energy and the maintenance of the general circulation: Tell’Us, v. 7, no. 2, p. 157–167.
- Marston, R.A., Mills, J.D., Wrazien, D.R., Bassett, B., and Splinter, D.K., 2005, Effects of Jackson lake dam on the Snake River and its floodplain, Grand Teton National Park, Wyoming, USA: Geomorphology, v. 71, no. 1–2, p. 79–98.
- Maupin, M.A., Kenny, J.F., Hutson, S.S., Lovelace, J.K., Barber, N.L., and Linsey, K.S., 2014, Estimated use of water in the United States in 2010: US Geological Survey.
- Myers, W.B., and Hamilton, W., 1964, The Hebgen Lake, Montana, earthquake of August 17, 1959: U.S. Geol. Surv. Prof. Pap., v. 435, p. 51.
- Oviatt, C.G., 2015, Chronology of Lake Bonneville, 30,000 to 10,000 yr B.P: Quat. Sci. Rev., v. 110, p. 166–171.
- Powell, J.W., 1879, Report on the lands of the arid region of the United States with a more detailed account of the land of Utah with maps: Monograph.
- Reed, J.C., Love, D., and Pierce, K., 2003, Creation of the Teton landscape: a geologic chronicle of Jackson Hole and the Teton Range: pubs.er.usgs.gov.
- Reese, R.S., 2014, Review of Aquifer Storage and Recovery in the Floridan Aquifer System of Southern Florida:
- Schele, L., Miller, M.E., Kerr, J., Coe, M.D., and Sano, E.J., 1992, The Blood of Kings: Dynasty and Ritual in Maya Art: George Braziller Inc.
- Seaber, P.R., Kapinos, F.P., and Knapp, G.L., 1987, Hydrologic unit maps:
- Solomon, S., 2011, Water: The Epic Struggle for Wealth, Power, and Civilization: Harper Perennial.
- Törnqvist, T.E., Wallace, D.J., Storms, J.E.A., Wallinga, J., Van Dam, R.L., Blaauw, M., Derksen, M.S., Klerks, C.J.W., Meijneken, C., and Snijders, E.M.A., 2008, Mississippi Delta subsidence primarily caused by compaction of Holocene strata: Nat. Geosci., v. 1, no. 3, p. 173–176.
- Turner, R.E., and Rabalais, N.N., 1991, Changes in Mississippi River water quality this century: Bioscience, v. 41, no. 3, p. 140–147.
- United States Geological Survey, 1967, The Amazon: Measuring a Mighty River: United States Geological Survey O-245-247.
- U.S. Environmental Protection Agency, 2014, Cyanobacteria/Cyanotoxins
- U.S. Geological Survey, 2012, Snowmelt - The Water Cycle, from USGS Water-Science School
- Utah/Nevada Draft Snake Valley Agreement, 2013
Component of the gravitational force which holds material on a slope.
Slope angle where shear forces and normal forces are equal.
QR Code generated with QRCode Monkey. All generated QR Codes are 100% free and can be used for whatever you want. This includes all commercial purposes.
A very fine-grained rock with very thin layering (fissile).
By Krishnavedala (Own work) [CC0], via Wikimedia Commons
By Tim Bertelink (Own work) [CC BY-SA 4.0], via Wikimedia Commons
Limestone made of primarily fine-grained calcite mud. Microscopic fossils are commonly present.
Extremely thin bedding in mudstones, a characteristic of shale.
Discernible layers of rock, typically from a sedimentary rock.
A specific layer of rock with identifiable properties.
A specific layer of rock formed by flowing fluid, either in the lowest part of the lower flow regime or lower part of the upper flow regime.
By Matt Affolter
Erosional rock face caused by sand abrasion.
Potentially extractible and valuable material, but unproven.
Subtle ridges formed in the upper flow regime on top of plane beds in the direction of flow.
Sedimentary layering disturbed by movement of organisms.
QR Code generated with QRCode Monkey. All generated QR Codes are 100% free and can be used for whatever you want. This includes all commercial purposes.
By Kraaiennest (Own work) [GFDL or CC BY-SA 4.0-3.0-2.5-2.0-1.0], via Wikimedia Commons
Rock with abraded surfaces formed in deserts.
Glaciers that form in cool or mountainous areas.
Oxidation that occurs in sulfide deposits which can concentrate valuable elements like copper.
A rock made primarily of clay.
By Brocken Inaglory (Own work) [CC BY-SA 3.0 or GFDL], via Wikimedia Commons
By Peter Kapitola (Own work) [CC BY-SA 2.5], via Wikimedia Commons
by Robert A. Rohde
Topographic prominence which sheds water into a specific drainage basin.
Upper layer of soil, made mainly out of organic material.
The process that turns non-desert land into desert.
Large surface mine with opening carved into the ground.
Groves scratched in rock by glacial action.
Data which is out of the ordinary and does not fit previous trends.
A specific type of sedimentary structure (ripples, plane beds, etc.) linked to a specific flow regime.
Lake that forms next to a glacier because of crustal loading.
The study of rock layers and their relationships to each other within a specific area.